10.2 Controls of Climate
R. Adam Dastrup and Laura J. Brown
Earth’s climate is composed of a complex system and interaction with other natural processes. To understand the current climate crisis, it is essential to understand the variables, controls, and systems that directly or indirectly affect it. (How Do Scientists Classify Different Types of Climate? | NOAA Climate.Gov, n.d.)
Latitude
The dominant control influencing the climate of a region is latitude because different latitudes receive different amounts of solar radiation. Depending on where the planet is on its revolution around the sun, the region between 23.5 degrees north and south receives the most solar radiation. Figure 10.1 illustrates the equinox when the Sun’s direct rays are striking at the equator.
The polar regions receive the least solar radiation because of the curvature of the Earth. The night lasts six months during the winter. Even in summer, the sun never rises high in the sky. Sunlight filters through a thick wedge of atmosphere, making the sunlight much less intense. The high albedo, because of ice and snow, reflects a good portion of the sun’s light. (Climate Systems and Change | Earth Science, n.d.)
Solar Energy Budget
The Earth’s climate is a solar-powered system. So to truly understand Earth’s climate, an understanding of the planet’s energy balance is essential. Consider Figure 10.2 an image from NASA on how incoming (yellow) and outcoming (red) energy sources of shortwave and longwave radiation achieve a net balance:
- At the surface
- Within the atmosphere
- At the top of the atmosphere

Next, let us note that Figure 10.2 represents average climate conditions over the entire Earth’s surface, and averaged over time. However, in reality, the incoming distribution of radiation varies in both space and time.
The dominant spatial variation occurs with latitude. There is more incoming solar radiation (insolation) arriving at the surface near the equator than near the poles. On average, roughly 30 percent of this incident radiation is reflected out to space by clouds and reflective surfaces of the Earth, such as ice and desert sand, leaving roughly 70 percent of the incoming solar radiation to be absorbed by the Earth’s surface. The portion reflected by clouds and by the surface also varies substantially with latitude, owing to the latitudinal variations in cloud and ice cover.
The intensity of insolation that the Earth receives is not constant over time. Over the lifetime of the sun, 4.6 billion years, energy output has varied considerably by roughly 30 percent. The amount of energy the planet receives from the sun is called the solar constant.
Even more dramatic changes in solar insolation take place on shorter timescales – the diurnal and annual timescales. These changes, however, do not have to do with the net output of the Sun, but rather the distribution of solar insolation over the Earth’s surface. This distribution is influenced by the Earth’s daily rotation about its axis, which, of course, leads to night and day, and the annual orbit of the Earth about the Sun, which leads to our seasons. While there is a small component of the seasonality associated with changes in the Earth-Sun distance during the course of the Earth’s annual orbit about the Sun (because of the slightly elliptical nature of the orbit), the primary reason for the seasons is the tilt of Earth’s rotation axis relative to the plane defined by the Earth and the Sun, which causes the Northern Hemisphere and Southern Hemisphere to be preferentially oriented either towards or away from the Sun, depending on the time of year.
The consequence of all of this is that the amount of shortwave radiation received from the Sun at the top of the Earth’s atmosphere varies as a function of both times of day and season. Subtle changes in the Earth’s orbital geometry (i.e., changes in the tilt of the axis, the degree of ellipticity of the orbit, and the slow precession of the orbit) are responsible for the coming and going of the ice ages over tens of thousands of years. (Overview of the Climate System (Part 2) | METEO 469: From Meteorology to Mitigation: Understanding Global Warming, n.d.)
Global Atmospheric Circulation
The imbalance of insolation results in greater heating at the Equator and in the tropics with the temperatures decreasing towards the polar regions as the insolation decreases. It is this unequal heating at the Earth’s surface that generates global atmospheric and oceanic circulation patterns that redistribute the warmth from the Tropics towards the polar regions. The warm land and water surfaces in the tropics heat the air above them, and that warm air will rise. Without the Earth’s axial tilt, its rotation and surface coverage of both land and water, a simple circulation pattern would develop due to air pressure and temperature differences (Figure 10.3a). The air at the equator would become very hot and rise, creating a band of low air pressure. When this warm air hit the upper atmosphere, where it could no longer rise, it would travel towards the north and south poles, cooling along the way. At the poles, the now cold, dense air would sink back to the surface, creating a region of high air pressure. At the surface the temperature and air pressure differences would drive the air back to the equator. So, in each hemisphere, there would be one circulation cell, redistributing heat.
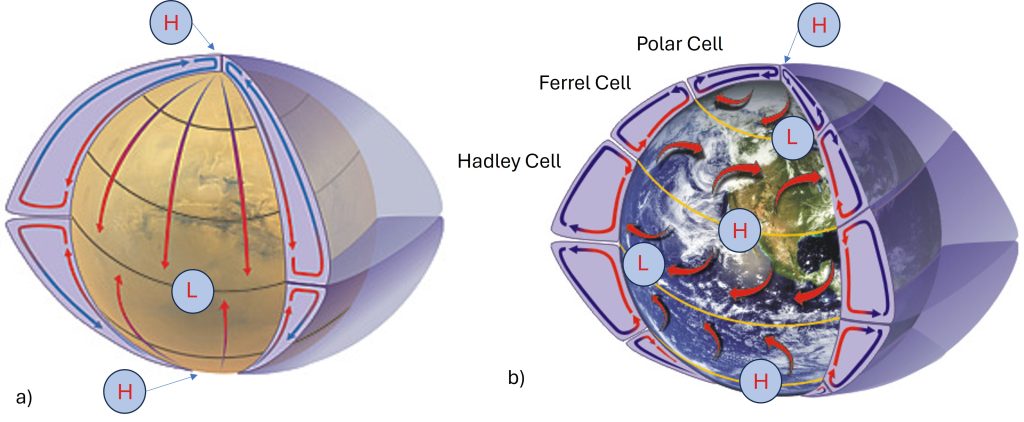
However, we have six circulation cells, three in each hemisphere (Figure 10.3b) due to unequal solar heating, land-water heating differences, and the Coriolis effect created by the Earth’s rotation. Starting at the equator and circulating in the low latitudes, between 0° and approximately 30º N and S, we have the Hadley Cell. Like the simplified circulation pattern, we have the warmed air rising from the equatorial region creating a band of low air pressure. As it rises, it begins to cool, and when it reaches the upper atmosphere, the air moves poleward. In the subtropics, at 30º, the air sinks back to the surface, creating a high pressure zone. Once the air reaches the surface, some of it travels back to the equator, where it is reheated, and the cycle begins again. The rest of it travels poleward in the Ferrel or Midlatitude Cell. In these midlatitude circulation cells, the air at the surface moves poleward and is forced to rise at the polar front, the boundary between the cold polar air and the warmer southern air (~60º). Once the rising air reaches the upper atmosphere, some of it travels back toward the subtropics, completing the Ferrel Cell (between 30º and 60º). In the high latitudes, we find the Polar Cell. This is the smallest of these circulation cells and is driven by extremely cold air that descends at the poles, creating areas of high air pressure. At the surface, the air moves outward from the poles towards 60º and the polar front. At the polar front, it rises, and some of it moves back toward the poles, where it eventually sinks, completing the Polar Cell (between 90º and 60º).
Intertropical Convergence Zone
The Intertropical Convergence Zone (ITCZ) is the low-pressure area near the equator in the boundary between the two Hadley Cells (Figure 10.4). The air rises so that it cools and condenses to create clouds and rain. The climate along the ITCZ is, therefore, warm and wet. Early mariners called this region the doldrums because their ships were often unable to sail because there were no steady winds.

The ITCZ migrates slightly with the season, following the relative motion of sunlight between the Tropic of Cancer and the Tropic of Capricorn. Land areas heat more quickly than the oceans. Because there are more land areas in the Northern Hemisphere, the ITCZ is influenced by the heating effect of the land. During the Northern Hemisphere’s summer, the ITCZ is approximately 5 degrees north of the equator, while in the winter, it shifts back and is approximately at the equator. As the ITCZ shifts, the dominant wind belts also shift slightly north in summer and south in winter, which causes the wet and dry seasons in this area.
Hadley Cell and Ferrell Cell Boundaries
At about 30º north and south, the air is relatively warm and dry because much of it came from the equator where it lost most of its moisture at the ITCZ. At this location, the air is descending, and sinking air warms and causes evaporation. Sunny conditions, with little precipitation, tend to occur. (Climate and Its Causes | Earth Science, n.d.)
Mariners named this region the Horse Latitudes. Sailing ships were sometimes delayed for so long by the lack of wind that they would run out of water and food for their livestock. Sailors tossed horses and other animals over the side after they died. Sailors sometimes did not make it either. (Earth Science | Simple Book Production, n.d.)
Prevailing Winds
The prevailing winds are the surface winds of the Hadley, Ferrell, and Polar cells. In the Hadley cell, the surface winds are deflected by the Coriolis Effect to the east creating the Northeasterly and Southeasterly Trade winds of the Tropics (Figure 10.5). In the Ferrel cell, the surface winds travelling poleward are again deflected by the Coriolis force, this time to the west, creating the Westerlies. In the Polar cell, the air moving outward from the poles is deflected to the east; these winds are the Easterlies. All these prevailing or dominant winds influence a region’s climate because they bring the weather conditions from the locations they come from. For example, in California, the predominant winds are the westerlies blowing in from the Pacific Ocean, which brings in relatively cool air in summer and relatively warm air in winter. Local winds also influence the local climate. For example, land breezes and sea breezes moderate coastal temperatures. (Earth Science | Simple Book Production, n.d.)
Landmasses
Location of Continents
When a location is near a large body of water, the local climate will be directly affected, creating a milder, maritime climate. Temperatures typically vary only slightly across the day and year. For a location to have an actual maritime climate, the winds must most often come off the sea. (Climate Systems and Change | Earth Science, n.d.)
When a location is not located near any large body of water, the region will typically experience a continental climate. A continental climate has more significant temperature differences between day and night and between summer and winter.
Altitude and Mountain Ranges
Atmospheric pressure and temperature decrease with altitude within the troposphere. The closer molecules are packed together, the more likely they are to collide. Collisions between molecules give off heat, which warms the air. At higher altitudes, the air is less dense, and air molecules are more spread out and less likely to collide. A location in the mountains has lower average temperatures than one at the base of the mountains. In Alberta, for example, the town of Banff (elevation 1,383 m) has an average daily mean temperature of 15ºC in July, while the city of Calgary (Elevation 1,045 m) mean July temperature is 17ºC.
Mountain ranges have two effects on the climate of the surrounding region. The first is something called the rain shadow effect, which brings warm, dry climate to the leeward side of a mountain range, as described in the Earth’s Atmosphere chapter. The second effect mountains have on climate systems is the ability to separate coastal regions from the rest of the continent. Since a maritime air mass may have trouble rising over a mountain range, the coastal area will have a maritime climate, but the inland area on the leeward side will have a continental climate.
Ocean Circulation Patterns
While we have focused primarily on the atmosphere thus far, the oceans, too, play a crucial role in relieving the radiation imbalance by transporting heat from lower to higher latitudes. The oceans also play a crucial role in both climate variability and climate change, as we will see. There are two primary components of ocean circulation. The first component is the horizontal circulation, characterized by wind-driven ocean gyres.

The major surface currents are associated with ocean gyres. These include the warm poleward western boundary currents such as the Gulf Stream, which is associated with the North Atlantic Gyre, and the Kuroshio Current associated with the North Pacific Gyre. These gyres also contain cold equatorward eastern boundary currents such as the Canary Current in the eastern North Atlantic and the California Current in the western North Atlantic. Similar current systems are found in the Southern Hemisphere. The horizontal patterns of ocean circulation are driven by the alternating patterns of wind as a function of latitude, and, in particular, by the tendency for westerly winds in mid-latitudes and easterly winds in the tropics, discussed above.
An essential additional mode of ocean circulation is the thermohaline circulation, which is sometimes referred to as the meridional overturning circulation or MOC. The circulation pattern is shown below. By contrast with the horizontal gyre circulations, the MOC can be viewed as a vertical circulation pattern associated with a tendency for sinking motion in the high-latitudes of the North Atlantic, and rising motion more broadly in the tropics and subtropics of the Indian and Pacific ocean. This circulation pattern is driven by contrasts in density, which are, in turn, primarily due to variations in both temperature and salinity (hence the term thermohaline). The sinking motion is associated with relatively cold, salty surface waters of the subpolar North Atlantic, and the rising motion with the relatively warm waters in the tropical and subtropical Pacific and Indian ocean.

The image above is a highly schematized and simple description of the actual vertical patterns of circulation in the ocean. Nonetheless, the conveyor belt is a useful mnemonic. The northward surface branch of this circulation pattern in the North Atlantic is sometimes erroneously called the Gulf Stream. The Gulf Stream, as discussed above, is part of the circulating waters of the wind-driven ocean gyre circulation. By contrast, the northward extension of the thermohaline circulation in the North Atlantic is rightfully referred to as the North Atlantic Drift. This current system represents a net transport of warm surface waters to higher latitudes in the North Atlantic and is also an essential means by which the climate system transports heat poleward from lower latitudes. Changes in this current system are speculated as having played a key role in past and potential future climate changes.
Air pressure and air temperature decrease with altitude. The closer molecules are packed together, the more likely they are to collide. Collisions between molecules give off heat, which warms the air. The air is less dense at higher altitudes, and air molecules are more spread out and less likely to collide. A location in the mountains has lower average temperatures than one at the base of the mountains. In Colorado, for example, Lakewood (5,640 feet) average annual temperature is 17 degrees Celsius (62 degrees Fahrenheit), while Climax Lake (11,300 feet) is 5.4 degrees Celsius (42 degrees Fahrenheit). (Climate and Its Causes | Earth Science, n.d.)
Composition of the Atmosphere
The troposphere is composed of nitrogen (78 percent), oxygen (21 percent), argon (less than 1 percent), and a variety of other gases. There are a few outliers, which include ozone and methane. Ozone is primarily found in the stratosphere that creates the ozone layer. Ozone is also found near the Earth’s surface from photochemical smog, from the emission of vehicles. Significant methane sources include the digestion of food from cattle, fracking of natural gas, coal mines, and deforestation. Because these sources have geographic locations where they occur, consistent sources on Earth’s surface can be found.
Greenhouse gases are composed of carbon dioxide, water vapor, methane, and some other gasses in smaller quantities. These gasses are essential for scientists to monitor, analyze, and understand because of their ability to trap radiation in the lower atmosphere. The greenhouse effect’s strength and intensity will depend on the atmosphere’s temperature and the number of greenhouse gases that the atmosphere holds.
The term “greenhouse effect” is an incorrect description of how energy is retained within the troposphere. Greenhouses warm the atmosphere within them by reducing airflow and circulation, not by “trapping heat.” Nevertheless, the Earth’s natural greenhouse effect is critical to supporting life. Human activities, such as burning fossil fuels and deforestation, have strengthened the natural greenhouse effect and causing the Earth’s atmosphere to warm over a noticeably short period.
Elevation
Atmospheric pressure and temperature decrease with altitude within the troposphere. The closer molecules are, the more likely they will collide into each other. Collisions between molecules give off energy and heat, which warms the surrounding atmosphere. At higher altitudes, the atmosphere is less dense, the air molecules are more spread out, and less likely to collide. On average, a location in the mountains has lower average temperatures than at the base of the mountains. Mountain ranges have two effects on the climate of the surrounding region. The first is called the rain shadow effect, which brings a warm, dry climate to the leeward side of a mountain range. The second effect mountains have on climate systems is the ability to separate coastal regions from the rest of the continent. Since a maritime air mass may have trouble rising over a mountain range, the coastal area will have a maritime climate, but the in-land area on the leeward side will have a continental climate. (Climate Systems and Change | Earth Science, n.d.)