3.4 The Impacts of Earthquakes
Steven Earle and Laura J. Brown
Some of the common impacts of earthquakes include structural damage to buildings, fires, damage to bridges, highways, pipelines and electrical transmission lines, initiation of slope failures, liquefaction, and tsunami. The types of impacts depend largely on where the earthquake is located: whether it is predominantly urban or rural, densely or sparsely populated, highly developed or underdeveloped, and of course, on the ability of the infrastructure to withstand shaking.
As we’ve seen from the example of the 1985 Mexico earthquake, the geological foundations on which structures are built can have a significant impact on earthquake shaking. When an earthquake happens, the seismic waves produced have a wide range of frequencies. The energy of the higher frequency waves tends to be absorbed by solid rock. In comparison, the lower frequency waves (with periods slower than one second) pass through the solid rock without being absorbed but are eventually absorbed and amplified by soft sediments. It is, therefore, very common to see much worse earthquake damage in areas underlain by soft sediments than in areas of solid rock. A good example of this is in the Oakland area near San Francisco, where parts of a two-layer highway built on soft sediments collapsed during the 1989 Loma Prieta earthquake (Figure 3.4.1).
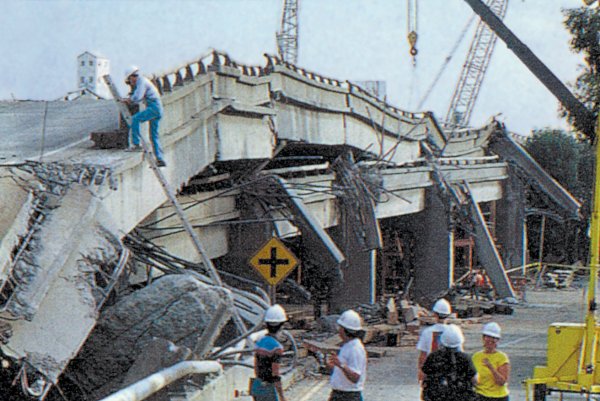
Building damage is also greatest in areas with soft sediments, and multi-storey buildings tend to be more seriously damaged than smaller ones. Buildings can be designed to withstand most earthquakes, and this practice is increasingly applied in earthquake-prone regions. Turkey is one such region, and even though Turkey had a relatively strong building code in the 1990s, adherence to the code was poor, as builders did whatever they could to save costs, including using inappropriate materials in concrete and reducing the amount of steel reinforcing. The result was that there were over 17,000 deaths in the 1999 M7.6 Izmit earthquake (Figure 3.4.2). After two devastating earthquakes that year, Turkish authorities strengthened the building code further. However, the new code has been applied only in a few regions, and enforcement of the code is still weak, as revealed by the amount of damage from an M7.1 earthquake in eastern Turkey in 2011.
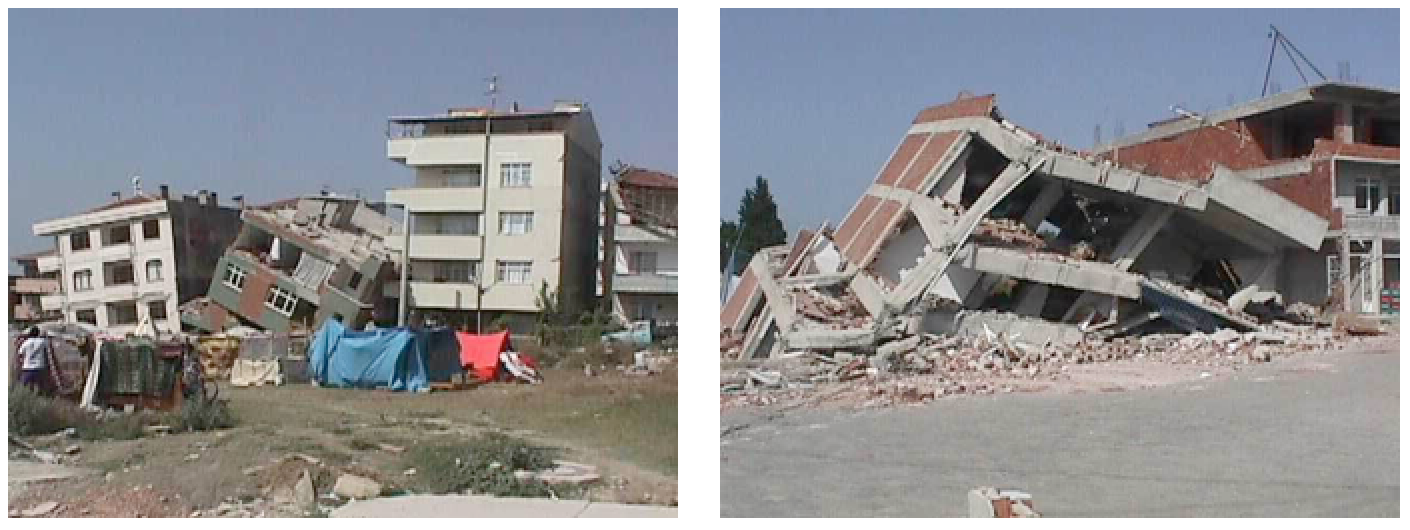
Fires are commonly associated with earthquakes because fuel pipelines rupture, and electrical lines are damaged when the ground shakes (Figure 3.4.3). Most of the damage in the great 1906 San Francisco earthquake was caused by massive fires in the downtown area of the city (Figure 11.4.4). Some 25,000 buildings were destroyed by those fires, which were fuelled by broken gas pipes. Fighting the fires was difficult because water mains had also ruptured. P-wave early warning systems can reduce the risk of fires if utility operators can reduce pipeline pressure and close electrical circuits.
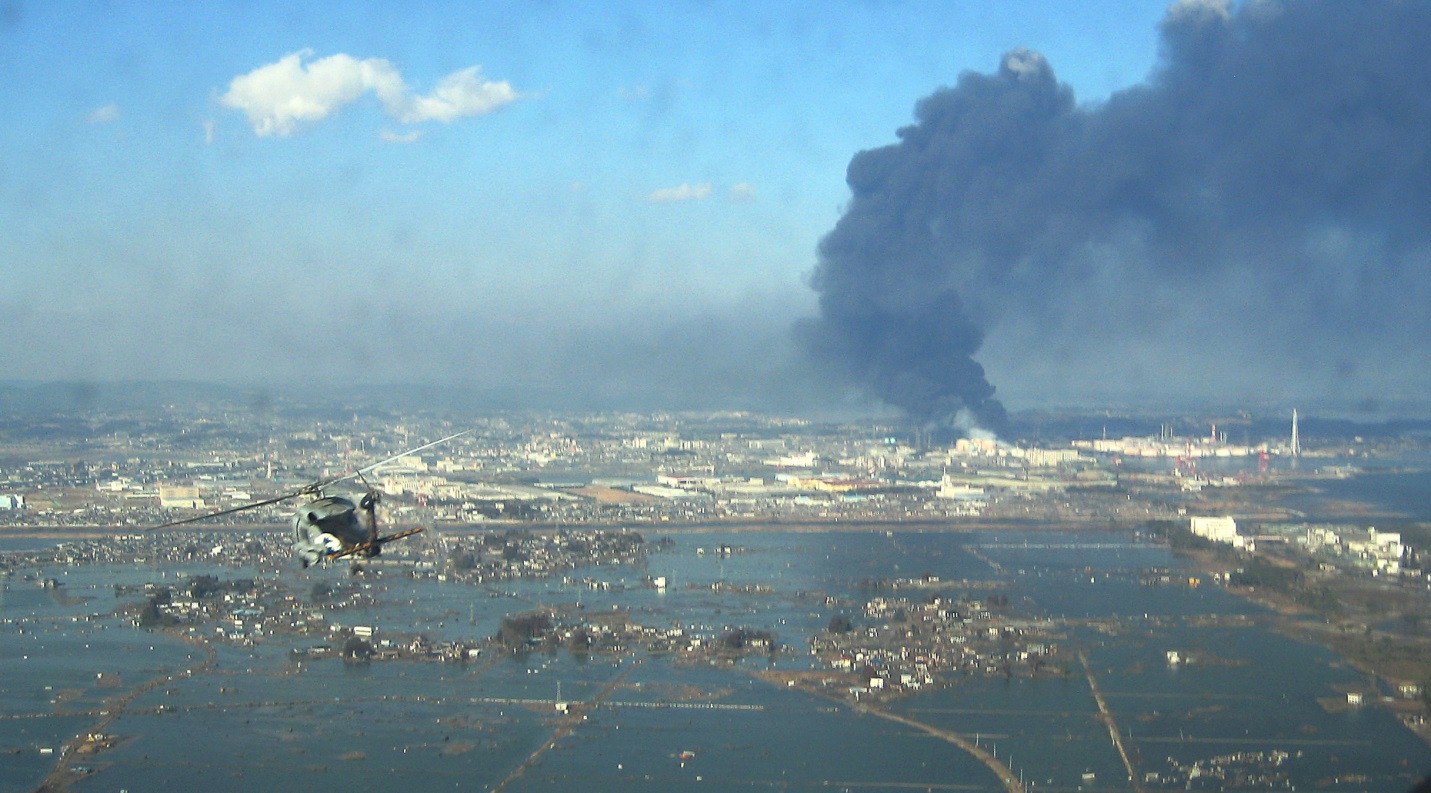
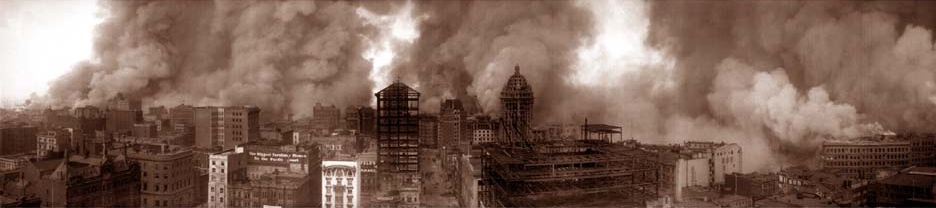
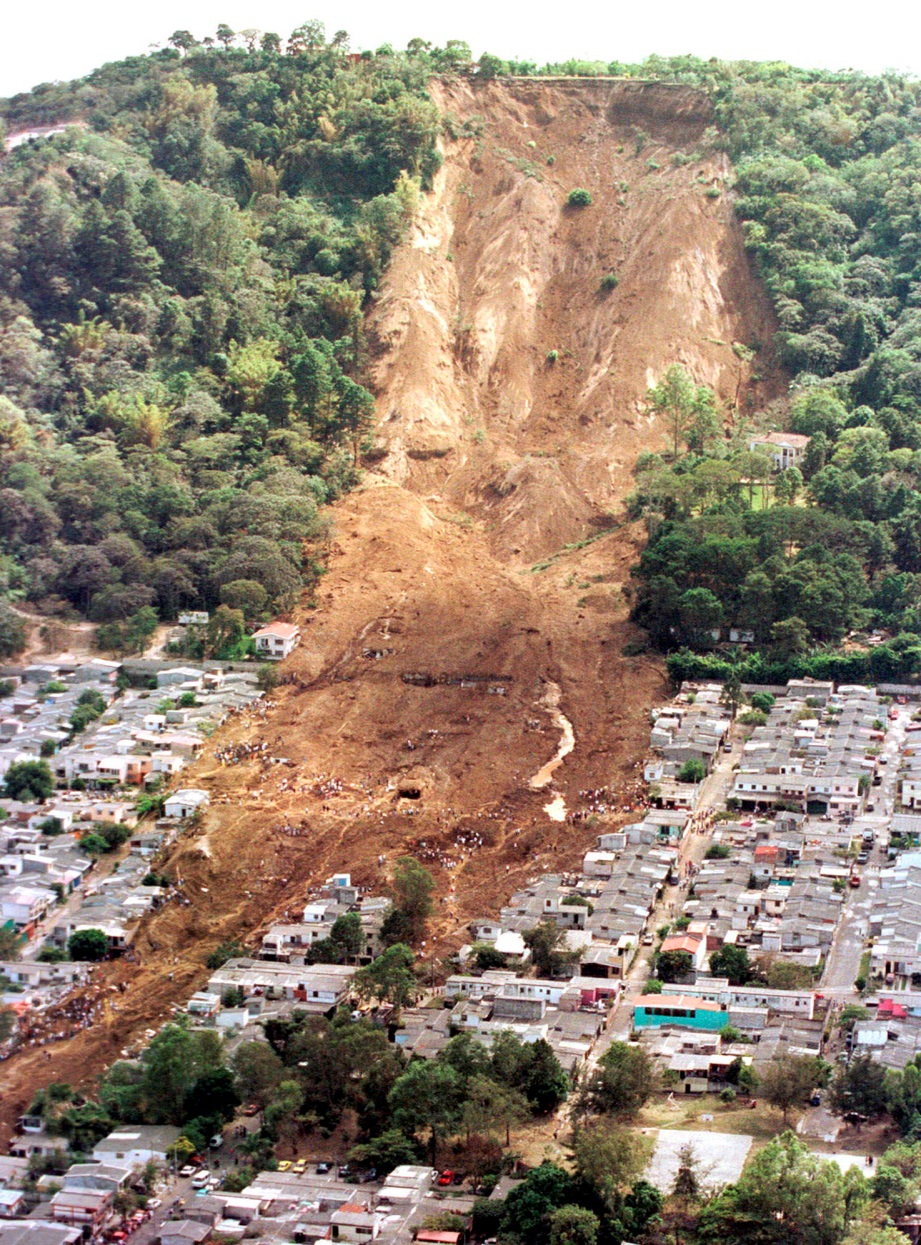
Earthquakes are important triggers for failures on slopes that are already weak. An example is the Las Colinas slide in the city of Santa Tecla, El Salvador, which was triggered by an M7.6 offshore earthquake in January 2001 (Figure 3.4.5).
Ground shaking during an earthquake can be enough to weaken rock and unconsolidated materials to the point of failure, but in many cases, the shaking also contributes to a process known as liquefaction, in which an otherwise solid body of sediment is transformed into a liquid mass that can flow. When water-saturated sediments are shaken, the grains become rearranged to the point where they no longer support one another. Instead, the water between the grains is holding them apart, and the material can flow. Liquefaction can lead to the collapse of buildings and other structures that might be otherwise undamaged. A good example is the collapse of apartment buildings during the 1964 Niigata earthquake (M7.6) in Japan (Figure 3.4.6). Liquefaction can also contribute to slope failures and fountains of sandy mud (sand volcanoes) in areas where loose saturated sand is beneath a layer of more cohesive clay.
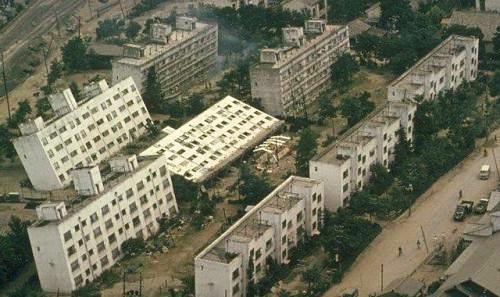
Parts of the Fraser River delta are prone to liquefaction-related damage because the region is characterized by a 2 metre to 3 metre thick layer of fluvial silt and clay over top of at least 10 metres of water-saturated fluvial sand (Figure 3.4.7). Under these conditions, it can be expected that seismic shaking will be amplified and that the sandy sediments will liquefy. This could lead to subsidence and tilting of buildings and to failure and sliding of the silt and clay layer. Current building-code regulations in the Fraser delta area require that measures be taken to strengthen the ground underneath multi-storey buildings before construction.
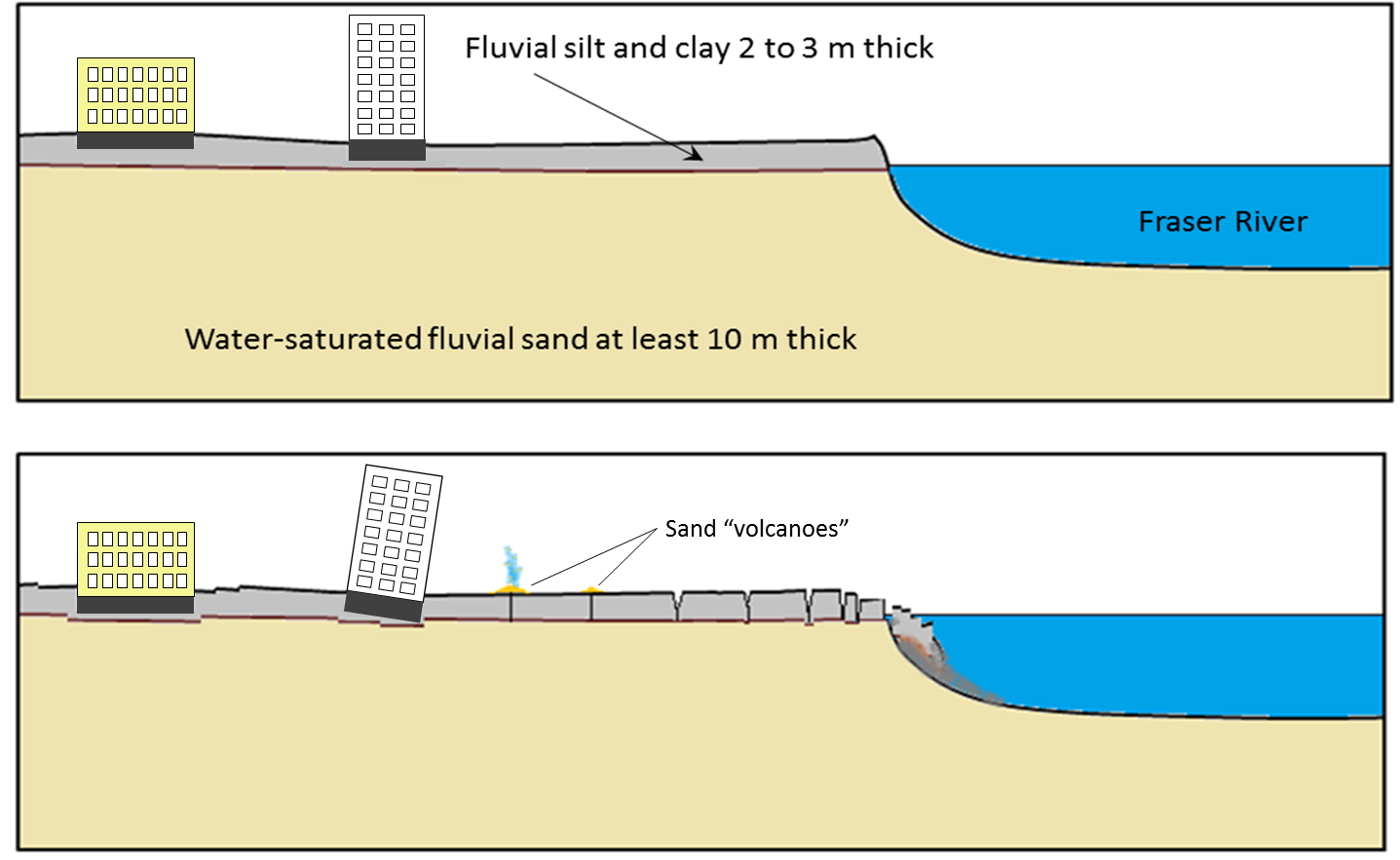
Exercise 3.4.1 Creating liquefaction and discovering the harmonic frequency
There are a few ways that you can demonstrate the process of liquefaction for yourself. The simplest is to go to a sandy beach (lake, ocean, or river) and find a place near the water’s edge where the sand is wet. This is best done with your shoes off, so let’s hope it’s not too cold! While standing in one place on a wet part of the beach, start moving your feet up and down at a frequency of about once per second. Within a few seconds, the previously firm sand will start to lose strength, and you’ll gradually sink in up to your ankles.
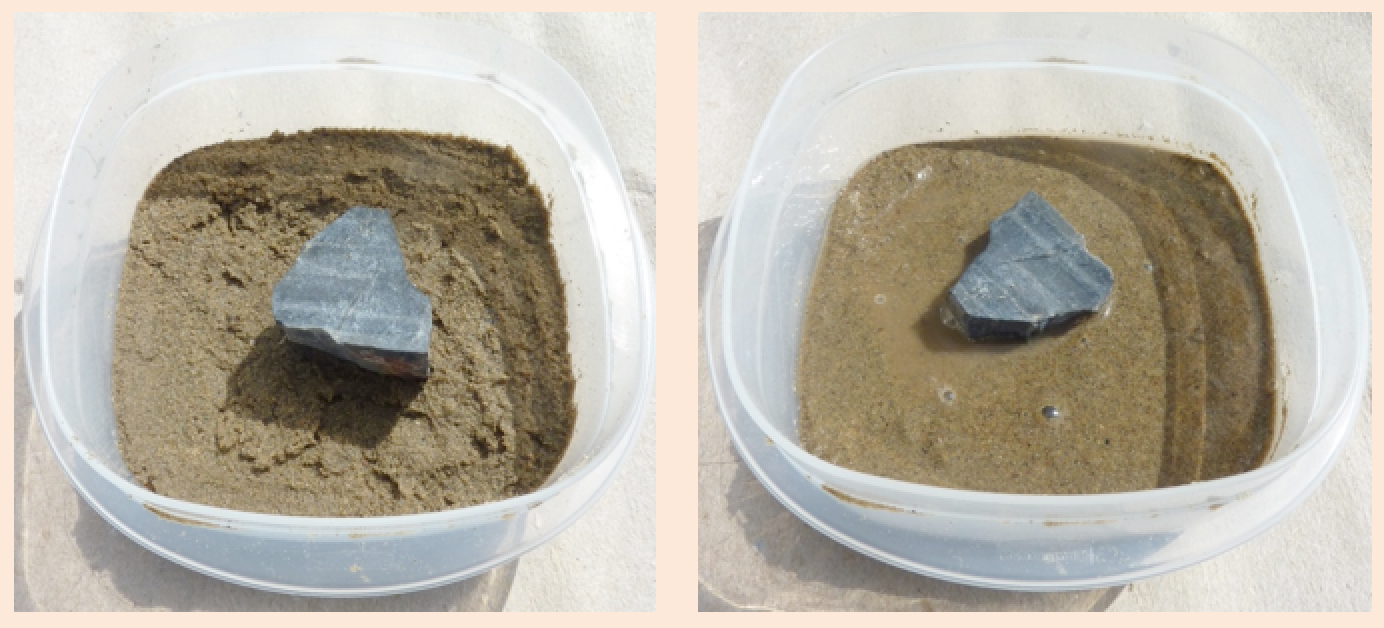
If you can’t get to a beach or the weather isn’t cooperating, put some sand (sandbox sand will do) into a small container, saturate it with water, and then pour the excess water off. You can shake it gently to get the water to separate and then pour the excess water away, and you may have to do that more than once. Place a small rock on the surface of the sand; it should sit there for hours without sinking in. Now, holding the container in one hand, gently thump the side or the bottom with your other hand, about twice a second. The rock should gradually sink in as the sand around it becomes liquefied.
As you were moving your feet up and down or thumping the pot, it’s likely that you soon discovered the most effective rate for getting the sand to liquefy; this would have been close to the natural harmonic frequency for that body of material. Stepping up and down as fast as you can (several times per second) on the wet beach would not have been effective, nor would you have achieved much by stepping once every several seconds. The body of sand vibrates most readily in response to shaking close to its natural harmonic frequency, and liquefaction is also most likely to occur at that frequency.
Earthquakes that take place beneath the ocean have the potential to generate a tsunami. (Tsunami is the Japanese word for harbour wave. It is the same in both singular and plural.) The most likely situation for a significant tsunami is a large (M7 or greater) subduction-related earthquake. As shown in Figure 3.4.9, during the time between earthquakes, the overriding plate becomes distorted by elastic deformation; it is squeezed laterally (Figure 3.4.9B) and pushed up.
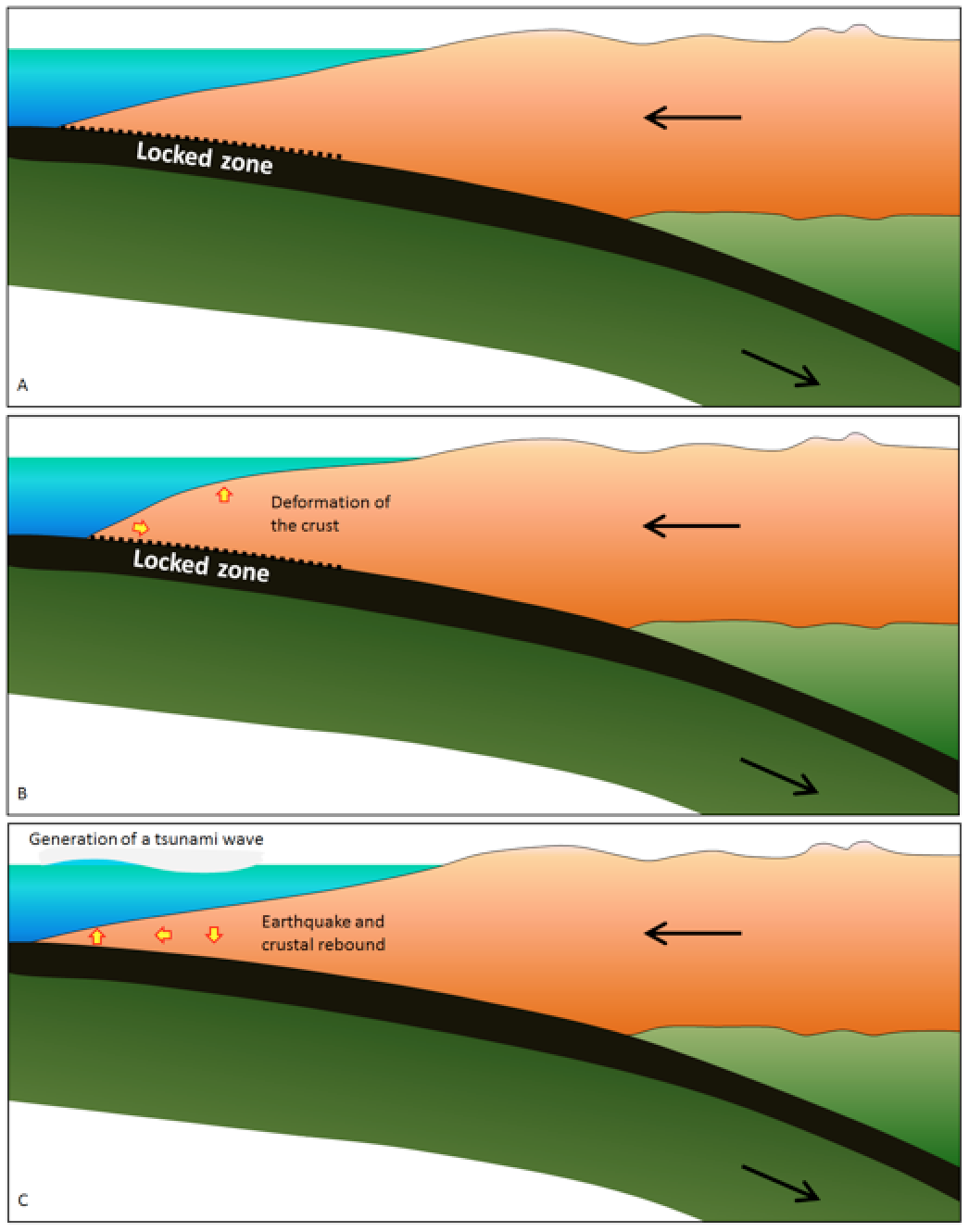
When an earthquake happens (Figure 3.4.9C), the plate rebounds, and there is both uplift and subsidence on the seafloor, in some cases by as much as several metres vertically over an area of thousands of square kilometres. This vertical motion is transmitted through the water column, where it generates a series of waves that then spread across the ocean.
Subduction earthquakes with a magnitude less than 7 do not typically generate significant tsunami because the seafloor’s vertical displacement is minimal. Sea-floor transform earthquakes, even large ones (M7 to M8), don’t typically generate tsunami either because the motion is mostly side to side, not vertical.
Tsunami waves travel at velocities of several hundred kilometres per hour and easily make it to the far side of an ocean at about the same time as a passenger jet. The simulated one shown in Figure 3.4.10 is similar to that created by the 1700 Cascadia earthquake off the coast of British Columbia, Washington, and Oregon, which was recorded in Japan nine hours later.
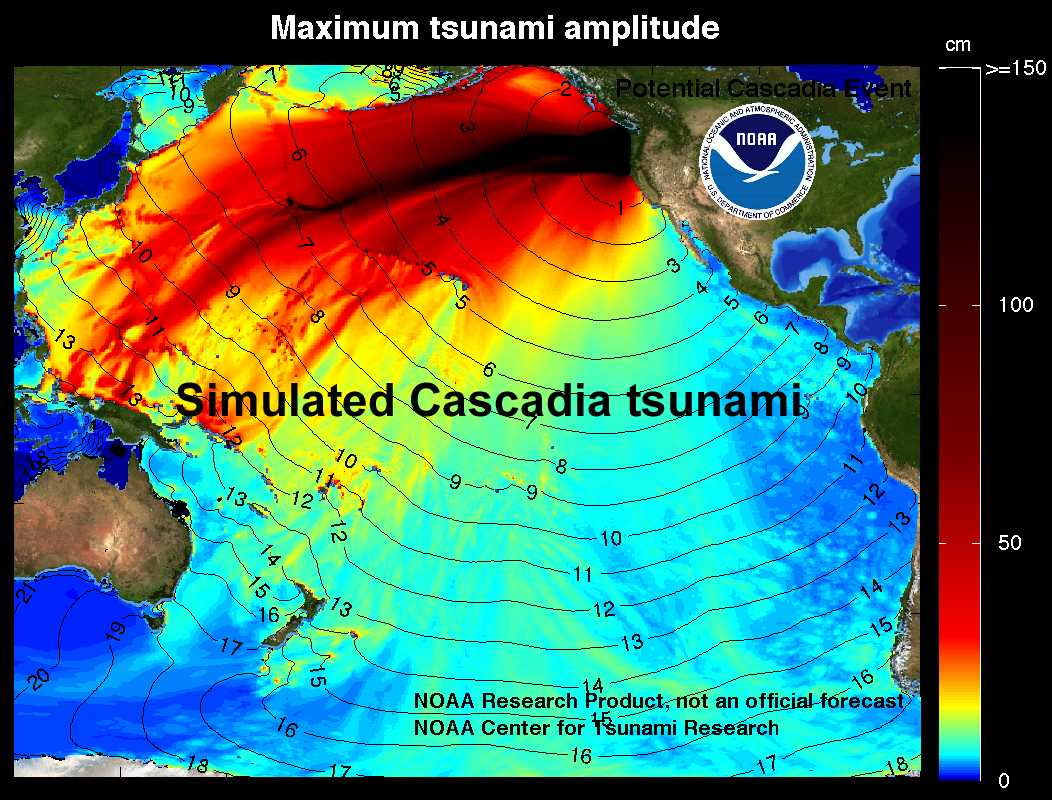
Tsunami are discussed further in Chapter 6.
Media Attributions
- Figure 3.4.1: “Cypress Collapsed” by the USGS. Public domain.
- Figure 3.4.2: “Izmit, Turkey Earthquake” by the USGS. Public domain.
- Figure 3.4.3: “SH-60B helicopter flies over Sendai” by the United States Navy. Public domain.
- Figure 3.4.4: “San Francisco Fire 1906” from the Library of Congress. Public domain.
- Figure 3.4.5: “ElSalvadorslide” by the USGS. Public domain.
- Figure 3.4.6: “Liquefaction at Niigata.” Public domain.
- Figures 3.4.7, 3.4.8, 3.4.9: © Steven Earle. CC BY.
- Figure 3.4.10: “Maximum Tsunami Amplitude” from NOAA/PMEL/Center for Tsunami Research. Public domain.