6.5 Factors That Control Slope Stability
Steven Earle and Laura J. Brown
Mass wasting happens because tectonic processes have created uplift. Erosion, driven by gravity, is the inevitable response to that uplift, and various types of erosion, including mass wasting, have created slopes in the uplifted regions. Slope stability is ultimately determined by two factors: the angle of the slope and the strength of the materials on it.
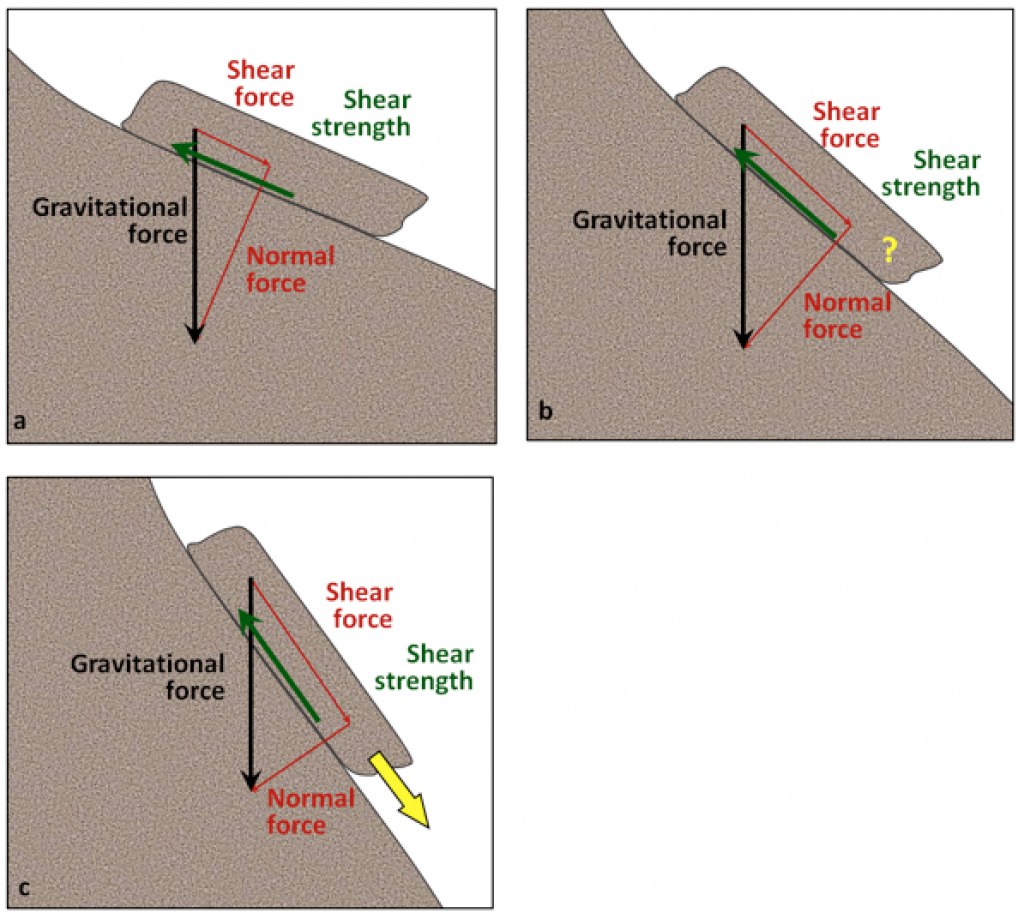
Figure 6.17 shows a block of rock situated on a rock slope. The block is being pulled toward Earth’s centre (vertically down) by gravity. We can split the vertical gravitational force into two components relative to the slope: one pushing the block down the slope (the shear force) and the other pushing into the slope (the normal force). The shear force, which wants to pull the block down the slope, has to overcome the strength of the connection between the block and the slope, which may be quite weak if the block has split away from the main body of rock or it may be very strong if the block is still a part of the rock. This is the shear strength, and in Figure 6.17a, it is greater than the shear force, so the block won’t move. In Figure 6.17b, the slope is steeper, and the shear force is approximately equal to the shear strength. The block may or may not move under these circumstances. In Figure 6.17c, the slope is even steeper than the other two, so the shear force is considerably greater than the shear strength, and the block will very likely move.
As already noted, slopes are created by uplift followed by erosion. Slopes tend to be quite steep in areas with relatively recent uplift (such as most of British Columbia and the western part of Alberta). This is especially true when glaciation occurs because glaciers in mountainous terrain create steep-sided valleys. In areas without recent uplift (such as central Canada), slopes are less steep because hundreds of millions of years of erosion (including mass wasting) have worn them down. However, as we’ll see, some mass wasting can happen even on relatively gentle slopes.
The strength of the materials on slopes can vary widely. Solid rocks tend to be strong, but there is a very wide range of rock strength. If we consider just the strength of the rocks and ignore issues like fracturing and layering, then most crystalline rocks—like granite, basalt, or gneiss—are very strong, while some metamorphic rocks—like schist—are moderately strong. Sedimentary rocks have variable strength. Dolostone and some limestone are strong, most sandstone and conglomerate are moderately strong, and some sandstone and all mudstones are quite weak.
Fractures, metamorphic foliation, or bedding can significantly reduce the strength of a body of rock. In the context of mass wasting, this is most critical if the planes of weakness are parallel to the slope and least critical if they are perpendicular to the slope. This is illustrated in Figure 6.18. The bedding is nearly perpendicular to the slope at locations A and B, and the situation is relatively stable. At location D, the bedding is nearly parallel to the slope, and the situation is quite unstable. The bedding is nearly horizontal at location C, and the stability is intermediate between the other two extremes.
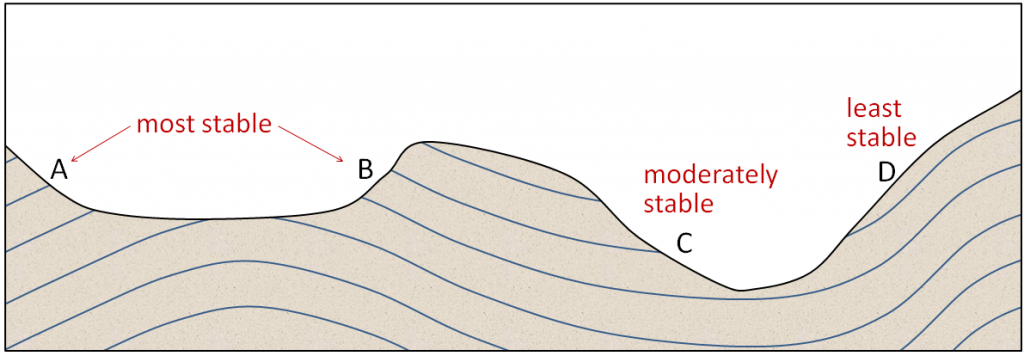
Internal variations in the composition and structure of rocks can significantly affect their strength. For example, Schist may have layers rich in sheet silicates (mica or chlorite), which will tend to be weaker than other layers. Some minerals are more susceptible to weathering than others, and the weathered products are commonly quite weak (e.g., the clay formed from feldspar). The side of Johnson Peak that failed in 1965 (Hope Slide) is made up of chlorite schist (metamorphosed seafloor basalt) with feldspar-bearing sills (they are evident within the inset area of Figure 6.2). The foliation and the sills are parallel to the steep slope. To begin with, the schist is relatively weak, and the feldspar in the sills, which has been altered to clay, makes it even weaker.
Unconsolidated sediments are generally weaker than sedimentary rocks because they are not cemented and, in most cases, have not been significantly compressed by overlying materials. This binding property of sediment is sometimes referred to as cohesion. Sand and silt tend to be particularly weak, clay is generally a little stronger, and sand mixed with clay can be stronger still. The deposits that make up the cliffs at Point Grey in Vancouver include sand, silt, and clay overlain by sand. Figure 6.19 (left) shows that the finer deposits are relatively strong (they maintain a steep slope). At the same time, the overlying sand is relatively weak and has a shallower slope that has recently failed. Glacial till—typically a mixture of clay, silt, sand, gravel, and larger clasts—forms and is compressed beneath tens to thousands of metres of glacial ice to be as strong as some sedimentary rock (Figure 6.19, right).
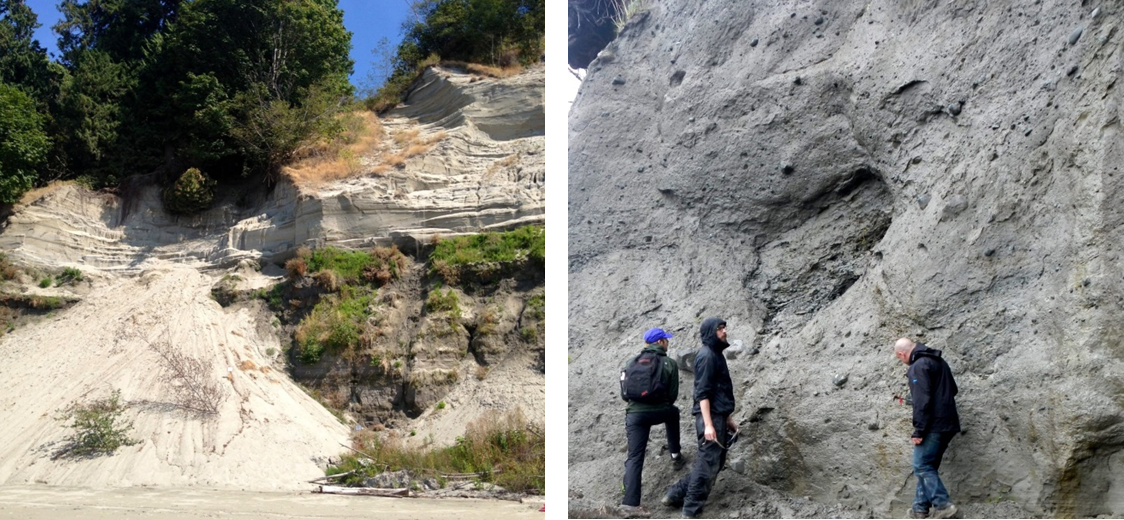
Apart from the type of material on a slope, the amount of water that the material contains is the most important factor controlling its strength. This is especially true for unconsolidated materials, like those shown in Figure 6.19, but it also applies to bodies of rock. Granular sediments, like the sand at Point Grey, have lots of spaces between the grains. Those spaces may be completely dry (filled only with air); or moist (often meaning that some spaces are water-filled, some grains have a film of water around them, and small amounts of water are present where grains are touching each other); or completely saturated (Figure 6.20). Unconsolidated sediments tend to be strongest when moist because the small amounts of water at the grain boundaries hold the grains together with surface tension. Dry sediments are held together only by the friction between grains, and if they are well sorted, or rounded, that cohesion is weak. Saturated sediments tend to be the weakest of all because the large amount of water actually pushes the grains apart, reducing the mount friction between grains. This is especially true if the water is under pressure.
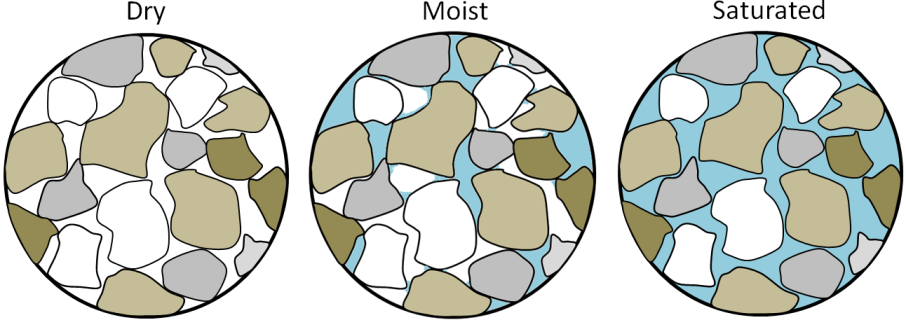
Exercise 6.3 Sand and water
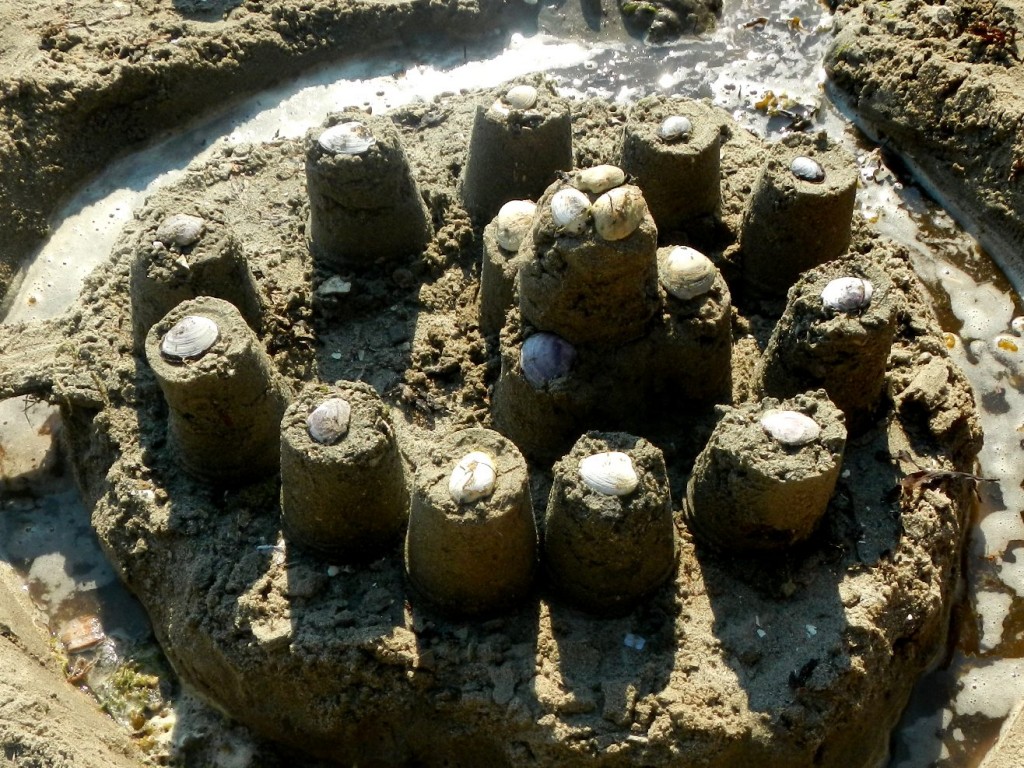
If you’ve ever been to the beach, you’ll already know that sand behaves differently when it’s dry than it does when it’s wet, but it’s worth taking a systematic look at the differences in its behaviour. Find about half a cup of clean, dry sand (or get some wet sand and dry it out), and then pour it from your hand onto a piece of paper. You should be able to make a cone-shaped pile that has a slope of around 30°. If you pour more sand on the pile, it will get bigger, but the slope should remain the same. Now add some water to the sand so that it is moist. An easy way to do this is to make it completely wet and then let the water drain away for a minute. You should be able to form this moist sand into a steep pile (with slopes of around 80°, Figure 6.21). Finally, put the same sand into a cup and fill the cup with water, so the sand is just covered. Swirl it around so that the sand remains in suspension, and then quickly tip it out onto a flat surface (best to do this outside). It should spread out over a wide area, forming a pile with a slope of only a few degrees.
Water will also reduce the strength of solid rock, especially if it has fractures, bedding planes, or clay-bearing zones. This effect is even more significant when the water is under pressure, which is why you’ll often see holes drilled into rocks on road cuts to relieve this pressure. One of the hypotheses advanced to explain the 1965 Hope Slide is that winter’s very cold conditions caused small springs in the lower part of the slope to freeze over, preventing water from flowing out. It is possible that water pressure gradually built up within the slope, weakening the rock mass to the extent that the shear strength was no longer greater than the shear force.
Water also has a particular effect on clay-bearing materials. All clay minerals will absorb some water, and this reduces their strength. The smectite clays (such as the bentonite used in clumping cat litter) can absorb a lot of water, and that water pushes the sheets apart at a molecular level and makes the mineral swell. Smectite that has expanded in this way has almost no strength; it is extremely slippery.
And finally, water can significantly increase the mass of the material on a slope, which increases the gravitational force pushing it down. A body of sediment with 25% porosity and is saturated with water weighs approximately 13% more than it does when it is completely dry, so the gravitational shear force is also 13% higher. In the situation shown in Figure 5.4.1b, a 13% increase in the shear force could easily be enough to tip the balance between shear force and shear strength.
Mass-Wasting Triggers
In the previous section, we discussed materials’ shear force and shear strength on slopes and factors that can reduce shear strength. Shear force is primarily related to slope angle, which does not change quickly. But shear strength can change quickly for various reasons, and events that lead to a rapid reduction in shear strength are considered triggers for mass wasting.
An increase in water content is the most common mass-wasting trigger. This can result from the rapid melting of snow or ice, heavy rain, or some type of event that changes the water flow pattern on the surface. Rapid melting can be caused by a dramatic increase in temperature (e.g., in spring or early summer) or almost instantaneously by a volcanic eruption. Heavy rains are typically related to major storms. Earthquakes can cause changes in water flow patterns, previous slope failures that dam up streams, or human structures that interfere with runoff (e.g., buildings, roads, or parking lots). An example of this is the deadly 2005 debris flow in North Vancouver (Figure 6.22). The 2005 failure occurred in an area that had failed previously. A report written in 1980 recommended that the municipal authorities and residents take steps to address surface and slope drainage issues. Little was done to improve the situation.
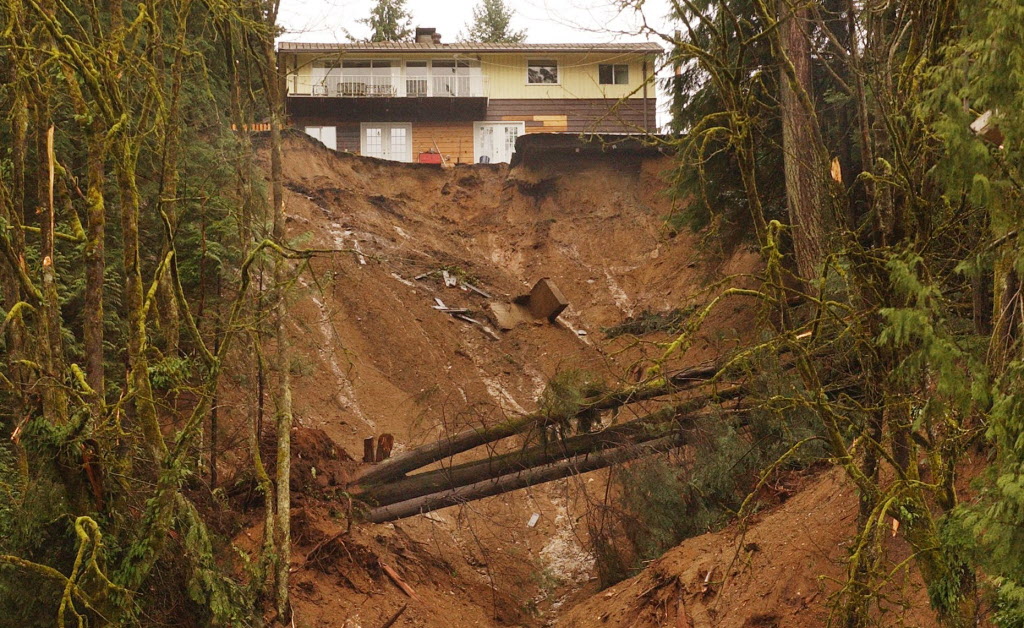
In some cases, a decrease in water content can lead to failure. This is most common with clean sand deposits (e.g., the upper layer in Figure 6.22 (left)), which lose strength when no water holds the grains together.
Freezing and thawing can also trigger some forms of mass wasting. More specifically, the thawing can release a block of rock that was attached to a slope by a film of ice.
One other process that can weaken a body of rock or sediment is shaking. The most obvious source of shaking is an earthquake, but shaking from highway or train traffic, construction, or mining will also do the job. Several deadly mass-wasting events (including snow avalanches) were triggered by the M7.8 earthquake in Nepal in April 2015.
Saturation with water and then seismic shaking led to the occurrence of thousands of slope failures in the Sapporo area of Hokkaido, Japan, in September 2018, as shown in Figure 6.23. The area was drenched with rain from tropical storm Jebi on September 4th. On September 6th, it was shaken by an M6.6 earthquake which triggered debris flows in the water-saturated volcanic materials on steep slopes. There were 41 deaths related to the slope failures.
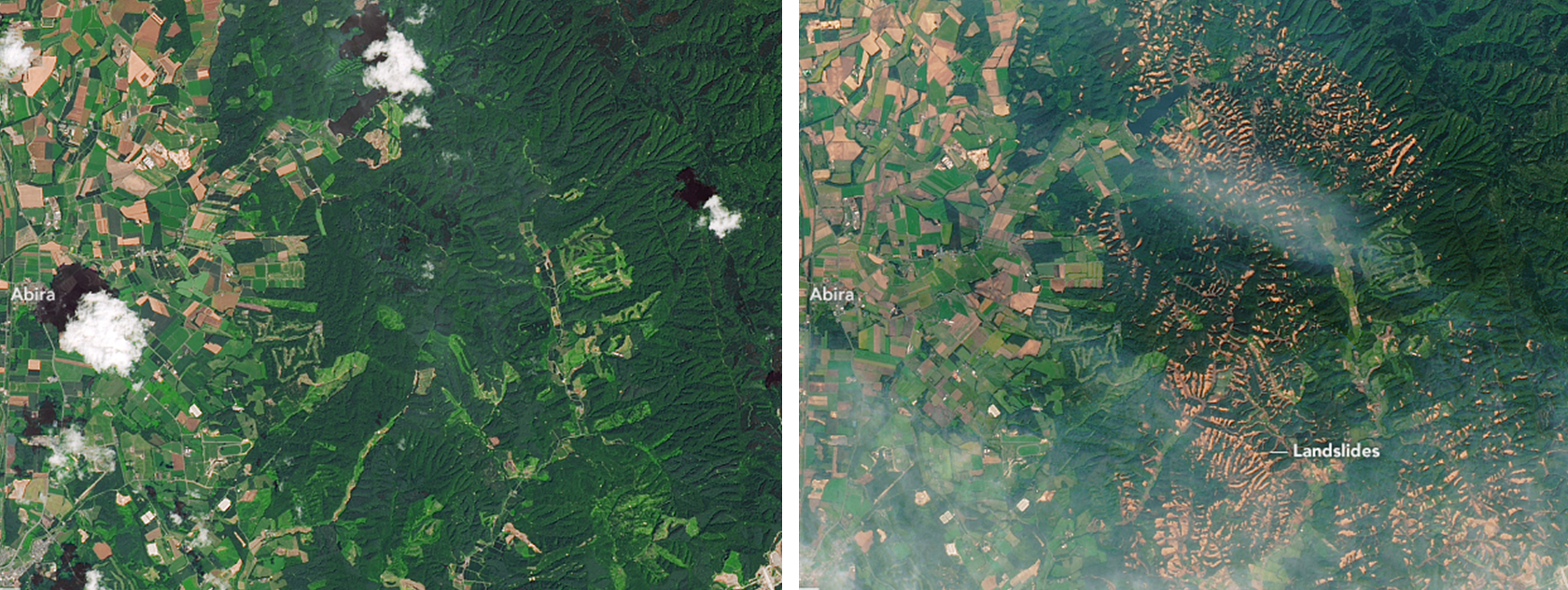
Media Attributions
- Figure 6.17, 6.18, 6.19, 6.20, and 6.21: © Steven Earle. CC BY.
- Figure 6.22: © The Province. Used with permission.
- Figure 6.23: “Landslides in Hokkaido” by Lauren Dauphin, NASA Earth Observatory. Public domain.