Action Potentials
Have you ever wondered how cells communicate with one another? Action potentials are one way this communication is accomplished. An action potential (AP) is a sudden change in membrane potential at a very specific point on the membrane in response to a stimulus. This section provides an overview of the action potential.
Learning Outcomes
In this section you will learn…
- Understand the concept of an action potential and how to represent it on a graph.
- Understand how voltage-gated sodium (Na+) and potassium (K+) channels create relative and absolute refractory periods.
- Explain the difference between “relative” and “absolute” refractory periods.
- Communicate the difference between an unmyelinated and myelinated neuron.
An action potential is the foundation of neuronal communication. It is an electrical signal that nervous tissue uses for communication. An action potential starts with a change in resting membrane potential, which we established to be −70 millivolts (mV) in the “Bioelectricity” section.
The following video provides an overview of the content you will encounter in this section. This introductory video uses the concepts you have learned from the bioelectricity section to explain how an AP occurs. Thus, this explanation is helpful to ‘bridge the gap’ between the two sections. The following concepts are covered: membrane potential at 0:50, depolarization at 1:15, repolarization at 1:40, refractory periods at 2:00, Sodium/Potassium Pump at 2:35 and local current at 3:05.
Steps to an Action Potential
Reaching Threshold
A stimulus from another cell or neuron can cause changes in resting membrane potential. However, not every change in resting membrane potential results in an action potential. A single stimulus OR a summation of stimuli must cause the resting membrane potential to change from −70mV to −55mv, known as “reaching threshold”.
Step 1: Depolarization
This results in voltage-gated sodium (Na+) channel opening in the nerve cell membrane. Because the concentration of Na+ is higher outside the cell than inside the cell, ions are driven by the concentration gradient to rush into the cell. Sodium is a positively charged ion, so this large influx of ions into the cell will change the voltage inside the cell to become less negative. This is known as depolarization, meaning the membrane potential moves toward zero.
Step 2: Repolarization
The concentration gradient for Na+ is so strong that it will continue to enter the cell until the membrane potential reaches approximately +30 mV. At this point, voltage-gated potassium channels open in the membrane. Now, potassium (K+) has a concentration gradient to leave the cell, taking a positive charge with it, the membrane potential begins to move back toward its resting voltage. This is called repolarization, meaning that the membrane voltage moves back toward the −70 mV value of the resting membrane potential.
Step 3: Hyperpolarization
Potassium ions actually reach equilibrium when the membrane voltage is below −70 mV. Since K+ channels are slightly delayed in closing, there is a slight period of overshoot where the membrane potential becomes more negative than −70mV. This period is called hyperpolarization.
The learning object below shows a graph of membrane potential (mV) relative to time. This graph is very useful because the various points are numbered and correspond to the steps shown below that explain what is happening in the cell that is leading to that specific membrane potential.
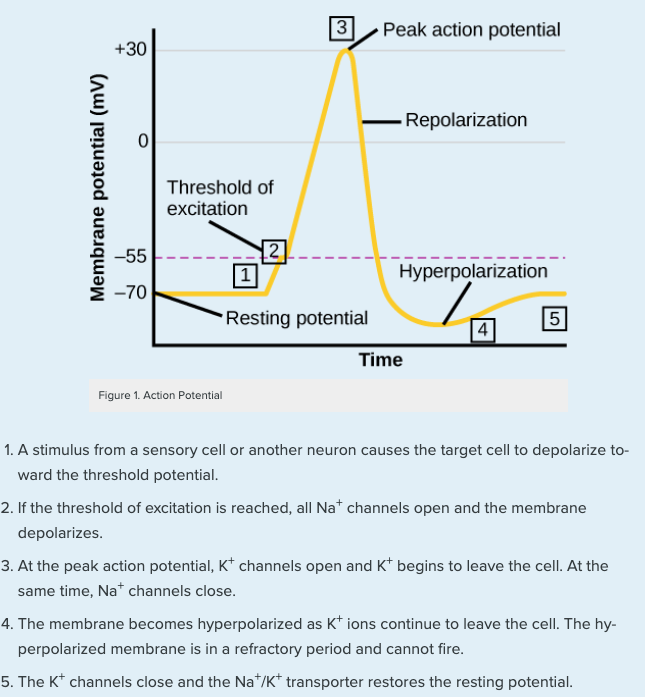
This short YouTube video provides an auditory and visual explanation of what we just covered with regard to action potentials. It begins by addressing what is occurring at the synapse to initiate an action potential in a neuron and then transitions to explain the various aspects of the action potential graph.
Are you interested in neurons? Check out this cool 15-second video of neurons firing under a microscope!
Test Your Knowledge
Thinking Beyond:
Our heart must contract rhythmically in order to pump blood throughout our body. Specialized cells in our heart, called Nodal Cells, have the capacity to spontaneously depolarize. This means that Nodal Cells do not require a stimulus to reach threshold. How is this possible? What do you suspect Nodal Cells are doing to spontaneously depolarize? Hint: How is threshold reached in a normal cell… How could you modify this normal cell to reach threshold on its own (i.e not require a stimulus)?
The following interactive content prompts you to order the sequential steps of an action potential in regard to ion channels and movement. This hands-on learning activity will help to solidify your understanding of ion movement during an action potential.
“All or Nothing”
Due to the threshold of −55mV, an action potential is considered an “all or nothing” event. This means that if threshold is not reached – meaning the stimulus doesn’t change resting membrane potential enough – then no action potential occurs.
On the other hand, a stimulus that might depolarize the membrane well past threshold will not make a “bigger” action potential. Either the membrane reaches the threshold and the steps of an AP take place, or the membrane does not reach the threshold and nothing else happens.
Tips From Past Students
You might be asking yourself: “If your nerves are trying to deliver a stronger stimulus (like a slap in the face versus a high five), does that not equate to a ‘bigger’ action potential?”
This is a common mistake, but remember that action potentials are simply an “all or nothing” event. In order to deliver a stimulus of varying levels, your nerves will change the frequency and pattern of action potentials. For example, if your nerves were carrying a stronger stimulus, they will initiate multiple action potentials more quickly, but the individual signals are not bigger.
In order to further understand the graph of an action potential, we must take a deeper look into voltage-gated Na+ and K+ channels.
Voltage-Gated Na+ Channels
The entire action potential occurs within approximately 2 milliseconds. Voltage-gated sodium channels open when the cell reaches threshold (−55mV) allowing sodium to rush into the cell. These channels are considered “fast” channels because they are open for a fraction of a millisecond. At the peak of depolarization (Step 3 in figure 1), these voltage-gated sodium channels close.
Voltage-Gated K+ Channels
Voltage-gated potassium channels are considered “slow” channels because they open and close slower than sodium channels. Potassium channels are triggered to open at −50mV, but because they are slow, they do not open until the peak of depolarization (Step #3 in figure 1). The inside of the cell has been flooded with sodium causing it to be highly positive, therefore, potassium ions will flow out of the cell (recall, this is the repolarization phase). These channels are slow to open as well as slow to close. The slow close causes the membrane potential to become hyperpolarized (more negative than resting membrane potential). Once potassium channels close, the membrane can return to the resting potential because of the ongoing activity of the non-gated channels and the Na+/K+ pumps.
Refractory Periods
While an action potential is in progress, another one cannot be initiated. This is called the refractory period. There are two phases of the refractory period: the absolute refractory period and the relative refractory period. With an absolute refractory period another action potential cannot start because voltage-gated sodium channels are inactive. No matter how large the voltage applied to the membrane is, an AP will not occur during this period. HOwever, during a relative refractory period, once voltage-gated sodium channels return to their resting conformation (less than −55mV), a new action potential can be started. However, potassium channels are still open and potassium is flowing out of the cell in an attempt to make the cell more negative and return to resting membrane potential. Therefore, a stronger stimulus is required to reach threshold (−55mV) and overcome potassium’s movement out of the cell. If the stimulus is not strong enough, any Na+ that enters the cell will not depolarize the cell, but will only keep the cell from hyperpolarizing.
The figure below labels the two refractory periods on the graph of an action potential. Comparing this graph to figure 1 can be useful to understand what is occurring in the neurons during the refractory periods.
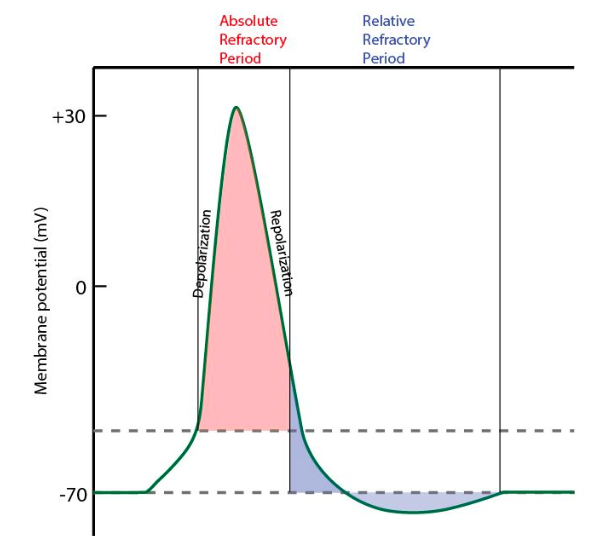
For an action potential to communicate information to another neuron, it must travel along the axon and reach the axon terminals where it can initiate the release of neurotransmitters. In order to understand the passing of this electrical impulse, we need to look at the structure of a neuron.
Neuron Structure
A neuron is an asymmetrical cell made up of a cell body (called the soma), the axon that propagates the action potential, and the axon terminal which passes the electrical signal to the next neuron. The figure below shows a labeled diagram of a neuron. Remember that action potentials travel from dendrites to the cell body, then along the axon.
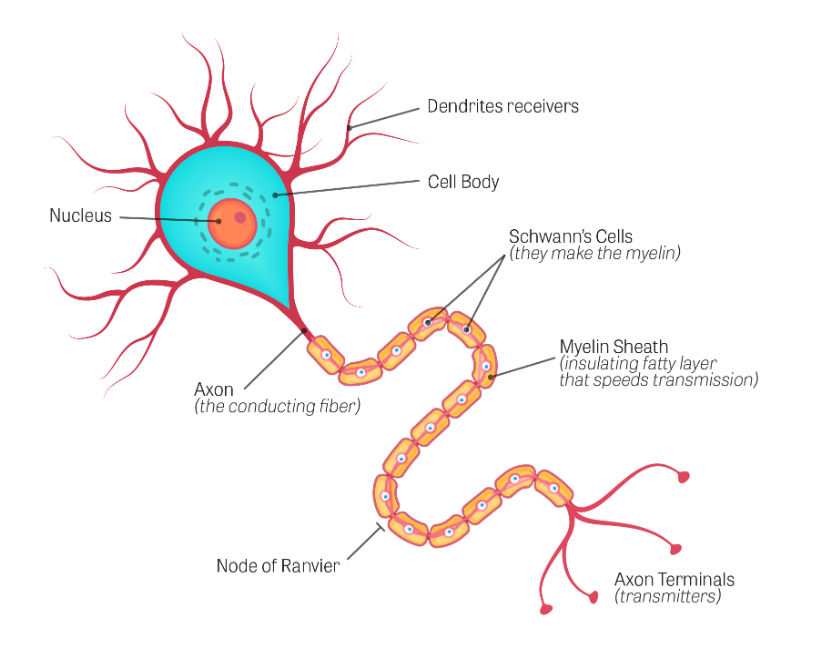
Did you know axons can be up to one meter in length?
An action potential is initiated at the beginning of the neuron axon. There is a high density of voltage-gated Na+ channels at the axon allowing that rapid depolarization to take place. As the AP moves along the cell membrane, the positive charge of Na+ rushing into the cell depolarizes a little more of the cell membrane. As that depolarization spreads, new voltage-gated Na+ channels open and more ions rush into the cell, spreading the depolarization a little farther. This can be termed “propagation” of the action potential along the length of the axon.
Tips From Past Students
You might be asking yourself: “If sodium is causing depolarization along the length of the cell membrane, what is stopping the action potential from traveling backward?”
Don’t forget that fast, voltage-gated sodium channels are inactivated at the peak of the depolarization (the absolute refractory period), and they cannot be opened again for a brief time. Because of this, depolarization spreading back toward previously opened channels has no effect. The action potential is, therefore “propagated” towards the end of the neuron (called the axon terminal) to the next neuron.
This is a classic example of cell asymmetry that allows for directionality of the action potential.
Speed of Conduction
There are a few factors that influence the speed of conduction of the AP down the axon. Myelination of neurons is critical to increase the speed of conduction of an action potential. Myelin is a sheath of fatty tissue that insulates the axon of a neuron. The myelin sheath wraps the axon in segments, broken up by Nodes of Ranvier, as shown in figure 5. Myelin is extremely important to help speed up the propagation of an action potential.
The diameter of the axon can also influence the speed of conduction. The Na+-based depolarization spreads faster down a wide axon than down a narrow one, because narrower axons have more resistance.
The following figure shows a zoomed-in image of an axon to exemplify the way that the myelin sheaths wrap around it. Keep in mind that the action potential jumps along the Nodes of Ranvier, which are the spaces between the myelin sheaths.
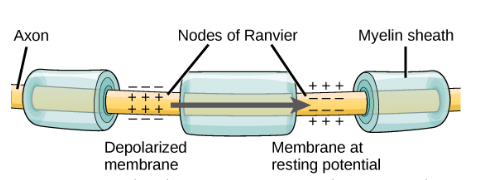
Propagation
Test Your Knowledge
Thinking Beyond:
What are two ways to improve the propagation of an AP? Hint: Think about the size of the axon. Think about the number of voltage-gated potassium and sodium channels. What could changing these things do to local current?
Interactive Overview
If you’d like to confirm your understanding of neuron structure, resting membrane potential, and action potentials, this learning object provides a great, short overview.
The following video provides a great summary of the action potentials section. It goes over the structure of a neuron (0:00-2:40), the steps of an action potential (2:40-4:25), refractory periods (4:25-5:20) and unidirectional movement (5:20-6:30).
Key Takeaways
Consider the following concepts to help guide your studies:
- An action potential is an ‘All or Nothing’ event
- Reaching threshold causes depolarization followed by repolarization and hyperpolarization
- By opening and closing voltage-gated sodium and potassium channels at specific times, we propagate an AP
- During an absolute refractory period, another AP cannot start because voltage-gated sodium channels are inactive
- During a relative refractory period, another AP can be generated if the stimulus is strong enough
- Action potentials travel from dendrites to the cell body, then along the axon
- Myelination is critical to increasing the speed of conduction of an AP
Subchapter Quiz
The questions below can be used to assess your knowledge within this chapter. There are five multiple-choice questions that you should attempt without referring to your notes. The questions will provide you with responses to your answers to guide your studying but should not be used as your only resource.
Media Attributions
- Private: Graph of an Action Potential © Lumen Learning is licensed under a All Rights Reserved license
- Private: Refractory Periods on an AP Graph © Brigham Young University is licensed under a All Rights Reserved license
- Private: Components of a Neuron © U.S. National Library of Medicine is licensed under a All Rights Reserved license
- Private: Myelination of an Axon © Charles Molnar and Jane Gair is licensed under a CC BY (Attribution) license
A change in membrane potential that passes along a neuron or muscle fiber to transmit an impulse.
The change in a cell's membrane potential that makes it more negative than the resting membrane potential.
The period in which another action potential cannot be generated even if another stimulus is applied.
The period during which a stimulus must be supermaximal to generate another action potential.
The propagation of action potentials along myelinated axons from one Node of Ranvier to the next, increasing the speed of conduction.
The propagation of an action potential along an unmyelinated axon.
Charge within the cell becomes more positive (upward slope of the curve).
Charge within the cell returns to being more negative (downward slope of the curve).
The formation and development of a myelin sheath around the axon of a neuron, which is affected by neuroglia, such as Schwann cells.