Vision
Learning Outcomes
In this section, you will learn…
- To identify anatomical structures of the eye.
- Descriptions of the function of photoreceptors in the transduction of light.
- To appreciate the brain’s role in visual processing.
Anatomy of the Eye and Vision[1]
The innermost layer of the eye is the neural tunic, or retina, which contains the nervous tissue responsible for photoreception. The eye is also divided into two cavities: the anterior cavity and the posterior cavity. The anterior cavity is the space between the cornea and lens, including the iris and ciliary body. It is filled with a watery fluid called the aqueous humor. The posterior cavity is the space behind the lens that extends to the posterior side of the interior eyeball, where the retina is located. The posterior cavity is filled with a more viscous fluid called the vitreous humor.
The retina is composed of several layers and contains specialized cells for the initial processing of visual stimuli. The photoreceptors (rods and cones) change their membrane potential when stimulated by light energy. The change in membrane potential alters the amount of neurotransmitter that the photoreceptor cells release onto bipolar cells in the outer synaptic layer. It is the bipolar cell in the retina that connects a photoreceptor to a retinal ganglion cell (RGC) in the inner synaptic layer. There, amacrine cells additionally contribute to retinal processing before an action potential is produced by the RGC. The axons of RGCs, which lie at the innermost layer of the retina, collect at the optic disc and leave the eye as the optic nerve, as see in figure 3. Because these axons pass through the retina, there are no photoreceptors at the very back of the eye, where the optic nerve begins. This creates a “blind spot” in the retina, and a corresponding blind spot in our visual field.
Note that the photoreceptors (cones and rods) in the retina are located behind the axons, RGCs, bipolar cells, and retinal blood vessels, shown in figure 2b below. A significant amount of light is absorbed by these structures before the light reaches the photoreceptors. However, in the centre of the retina, called the fovea, there are only cones cells with very few structures absorbing light in front of them. Therefore, the fovea is where the least amount of incoming light is absorbed by other retinal structures, as see in figure 1 below. Therefore, visual acuity, or the sharpness of vision, is greatest at the fovea. This is because the fovea is where the least amount of incoming light is absorbed by other retinal structures, shown in figure 1 below.
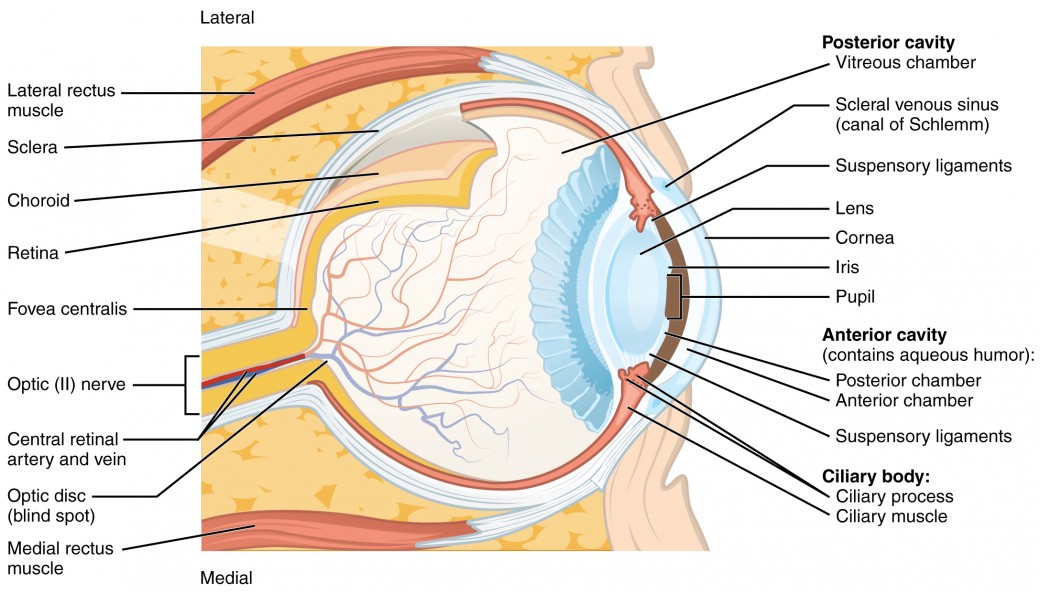
We mention ganglion cells numerous times throughout this chapter, however, there are actually three different types of ganglion cells, all with different functions. P-type retinal ganglion cells make up 55% of all retinal ganglion cells. These neurons project onto the parvocellular layers of the LGN. P-type retinal ganglion cells have intermediate conduction speed and provide information regarding wavelengths of light, and therefore colour. M-type retinal ganglion cells only make up 5% of ganglion cells, but they have fast conduction speed and project onto the magnocellular layers of the LGN. M-type retinal ganglion cells provide information regarding the intensity of light, more specifically from rapid eye movement and change in light intensity. The last type of retinal ganglion cell is called W-type. They have a slow speed of conduction and will provided information regarding intensity, mainly regarding direction movement. Both W- and M-type ganglion cells receive information from rods, as they are mainly associated with intensity. Contrast this to P-type neurons which are associated with colour and therefore cones.
Tips From Past Students
In the following video, the concept of phototransduction 3:59-4:57 and the arrangement of retinal neurons will be explained 0:20-2:04. This will help you to appreciate the fact that light must travel past all the neurons of the retina to reach the photoreceptors 1:00-2:04. Since the signal that are created by phototransduction travels through these retinal neurons, it means that the light coming into the eye will be going in the opposite direction than the signals. The video will also examine the important functions of amacrine and horizontal cells 1:44-2:04, as well as the differences between rods and cones 2:05-2:42. The important differences to note are their distribution in the retina 2:43-3:58 and why cones in the retina have the highest visual acuity 3:02-3:30.
“The Retinal Layers & Phototransduction” by Raechel Soicher is All Rights Reserved.
Structure and Function of the Eye [2]
The photoreceptors of the eye, where transduction of light into nervous impulses occurs, are located in the innermost part of the retina. This means that light will have to pass through several ocular structures to reach its receptor, as seen in figure 2b. The cornea, the front transparent layer of the eye, and the crystalline lens, a transparent convex structure behind the cornea, both refract (bend) light to focus the image on the retina. The iris, which is the coloured part of the eye, is a circular muscular ring lying between the lens and cornea that regulates the amount of light entering the eye. In conditions of high ambient light, the iris contracts, reducing the size of the pupil at its center, allowing less light to enter the eye. In conditions of low light, the iris dilates, and the pupil enlarges, increasing the amount of light let into the eye.
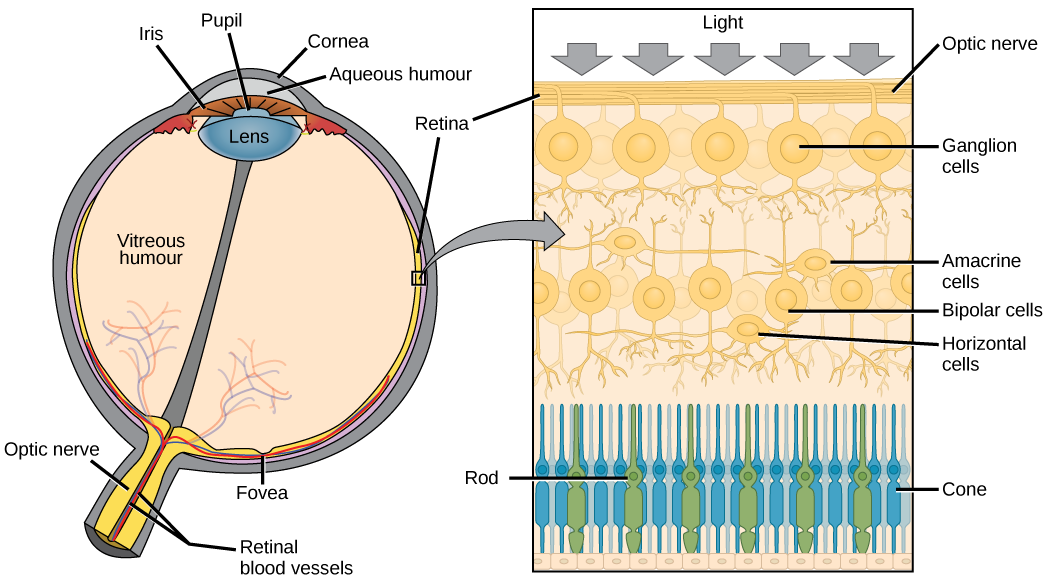
Testing Your Knowledge
Thinking beyond:
Let’s see how your knowledge regarding photoreceptors the arrangement of neurons in the retina can be applied to these questions. Why would it be advantageous to have the photoreceptors located behind all other retinal neurons? And on that same point, why wouldn’t the retinal neurons that are in front of the photoreceptors be activated by photons of light? Hint: Consider the sensitivity of these photoreceptors, and what the main function of those retinal neurons in front of it are.
Photoreceptors
Photoreceptors have two parts, the inner segment and the outer segment, shown in figure 3. The inner segment contains the nucleus and other common organelles of a cell, whereas the outer segment is a specialized region in which photoreception takes place. There are two types of photoreceptors—rods and cones—which differ in the shape of their outer segment. The rod-shaped outer segments of the rod photoreceptor contain a stack of membrane-bound discs that contain the photosensitive pigment rhodopsin and are located in the outer edges of the retina. They detect dim light and are used primarily for peripheral and night-time vision. The cone-shaped outer segments of the cone photoreceptor contain their photosensitive pigments in infoldings of the cell membrane and are located near the center of the retina. There are three cone photopigments, called opsins, which are each most sensitive to a range of wavelengths on the visual spectrum. The wavelength of visible light determines its color. The pigments in human eyes are specialized in perceiving three different colors: red, green, and blue.
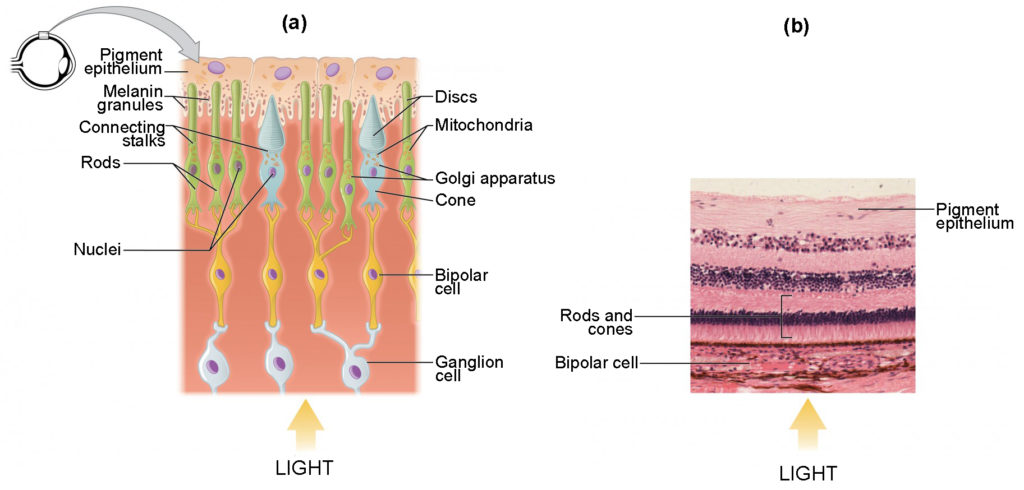
Retinal Processing
Visual signals leave the cones and rods, travel to the bipolar cells, and then to ganglion cells. A large degree of processing of visual information occurs in the retina itself, before visual information is sent to the brain.
Photoreceptors in the retina undergo tonic activity, meaning they are constitutively active, even when not stimulated by light. In neurons that exhibit tonic activity, the absence of stimuli maintains a firing rate at a baseline, some stimuli increase the firing rate from the baseline, and other stimuli decrease the firing rate. In the absence of light, the “on” bipolar neurons that connect rods and cones to ganglion cells are continuously and actively inhibited by the rods and cones. (Knowing this, how do you think this would work in the case of “off” bipolar cells?) Exposure of the retina to light hyperpolarizes the rods and cones and removes their inhibition of bipolar cells. The now active bipolar cells in turn stimulate the ganglion cells, which send action potentials along their axons (which leave the eye as the optic nerve). Thus, the visual system relies on a change in retinal activity, rather than the absence or presence of activity, to encode visual signals for the brain. Sometimes horizontal cells carry signals from one rod or cone to other photoreceptors and to several bipolar cells. When a rod or cone stimulates a horizontal cell, the horizontal cell inhibits more distant photoreceptors and bipolar cells, creating lateral inhibition. This inhibition sharpens edges and enhances contrast in the images by making regions receiving light appear lighter and dark surroundings appear darker. Amacrine cells can distribute information from one bipolar cell to many ganglion cells.
Ever wonder why you see a blue circle after looking at the sun? Try this exercise! Look fixedly at figure 8 for about 45 seconds. Then quickly shift your gaze to a sheet of blank white paper or a white wall. You should see an afterimage of the Norwegian flag in its correct colours. At this point, close your eyes for a moment, then reopen them, looking again at the white paper or wall; the afterimage of the flag should continue to appear as red, white, and blue. What causes this?
According to an explanation called opponent-process theory, as you gazed fixedly at the green, black, and yellow flag, your retinal ganglion cells that respond positively to green, black, and yellow increased their firing dramatically. When you shifted your gaze to the neutral white ground, these ganglion cells abruptly decreased their activity and the brain interpreted this abrupt downshift as if the ganglion cells were responding now to their “opponent” colours: red, white, and blue, respectively, in the visual field.
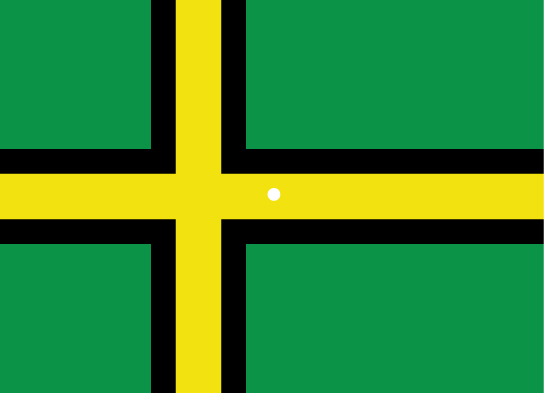
When light hits the rod cell it becomes hyperpolarized and a decrease in glutamate release occurs from the photoreceptor. These photoreceptors are connected to bipolar cells. Bipolar cells have receptive fields that are arranged in a centre-surround orientation of photoreceptors. Both the centre and surrounding area can either be off (meaning the bipolar cell will get hyperpolarized if light hits them) or on (meaning the bipolar cell will be depolarized when light hits them). On-centre off-surround receptive fields will depolarize the bipolar cell when photons exclusively hyperpolarize photoreceptors in the centre or when there are more photons hitting the centre than the surround. When photons hit surrounding rods alone the bipolar cell will be hyperpolarized. Photons equal activating both the centre and surround results in the bipolar cell remaining at its resting membrane potential, this is all a phenomenon known as centre-surround antagonization. On the other hand, off-centre on-surround receptive fields cause the bipolar cell to depolarize when light hits the surrounding rod cells, and hyperpolarize when light hits the centre of the receptive field. Both of these effects on the bipolar cell will result in neurotransmitter release onto the connecting ganglion cells, which will change its action potential frequency and pattern in order to relay the signal to the brain.
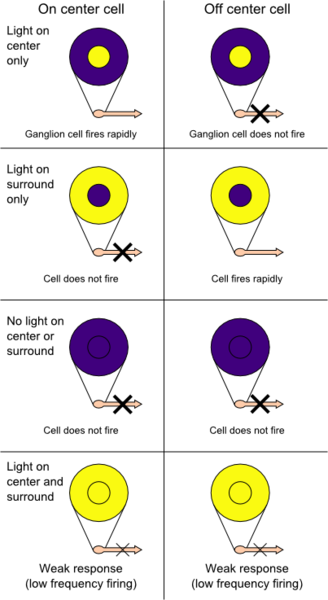
Having troubles visualizing how on centre surround systems exist in the retina? Here’s a cross-sectional picture that may help.
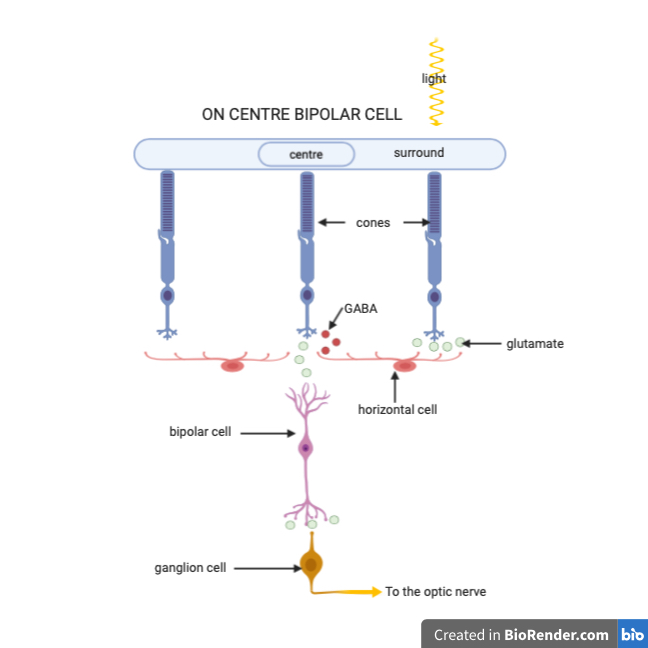
While this is vastly simplified, it does help grasp the concept of how the organization of the receptive field creates centres and surrounds.
Testing Your Knowledge
Thinking beyond:
With your knowledge of retinal neurons let’s answer the following questions. How could two photoreceptors cause contradicting activity in one downstream neuron? One causes activation and the other causes inhibition. Hint: Remember that photoreceptors themselves all respond in the identical way, with a decreased release of glutamate.
The Biochemical Level of Transduction[3]
Rods and cones transduce the signal of light into a nerve impulse. Both rods and cones contain photopigments. In vertebrates, the main photopigment, rhodopsin, has two parts. An opsin, which is a membrane protein, and retinal—a molecule that absorbs light. When light hits a photoreceptor, it causes a conformation change in the retinal, altering its structure from a bent (cis) form of the molecule to its linear (trans) isomer. This isomerization (conformational change) of retinal activates the rhodopsin, starting a cascade of events that ends with the closing of Na+ channels in the membrane of the photoreceptor as seen in figure 6. Thus, unlike most other sensory neurons (which become depolarized by exposure to a stimulus) visual receptors become hyperpolarized and thus driven away from threshold.
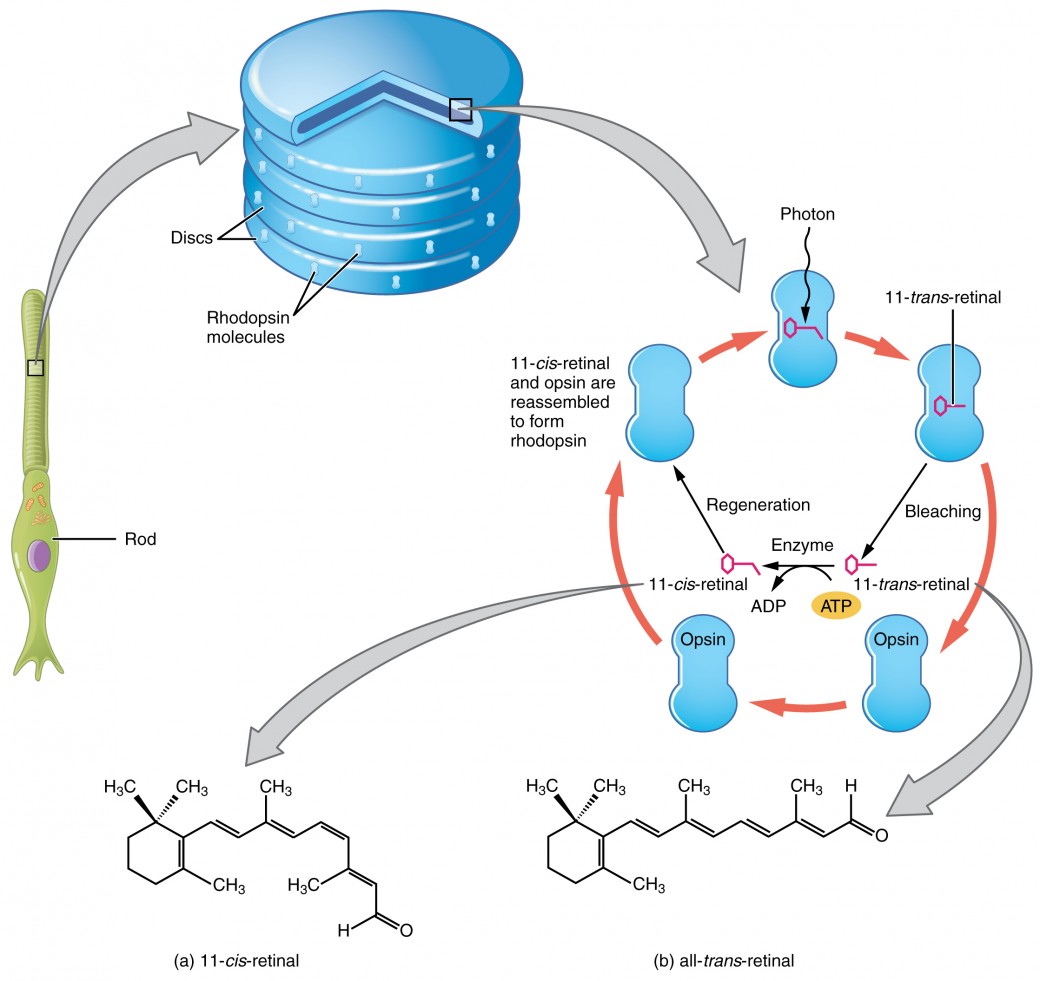
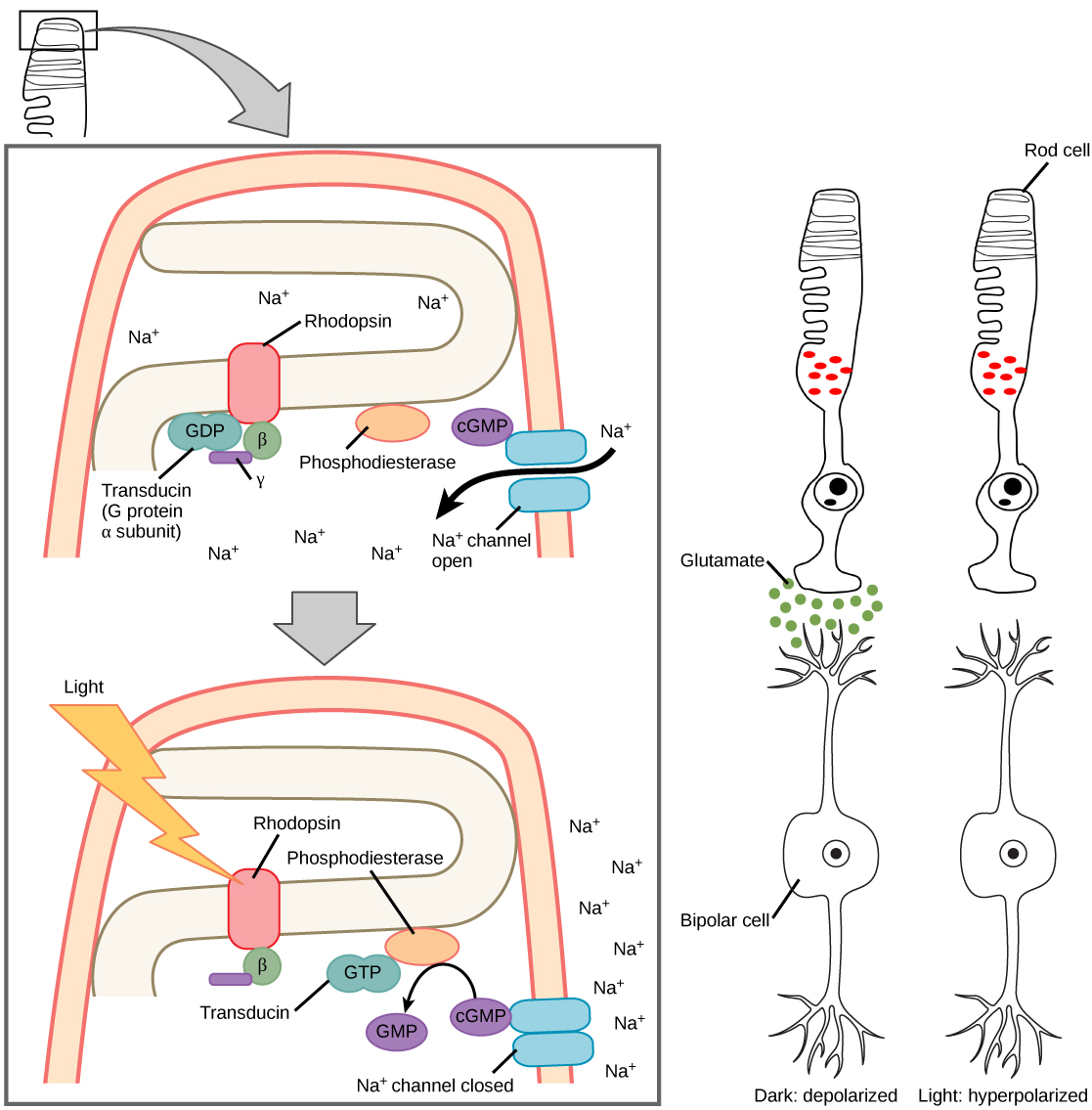
After the membrane is hyperpolarized, there is a decrease in glutamate release. If low concentration of glutamate arrives at an “off” bipolar cell next, it causes hyperpolarization because we are opening a fewer amount of sodium channels. If low concentration of glutamate arrives at an “on” bipolar cell next, it causes depolarization because we are decreasing the number of potassium and chloride channels that are open.
Khan Academy has another great video on phototransduction. They give an explanation of the cascade of events within the photoreceptor 0:05-7:19 that results in closure of Na+ channels 7:20-8:44. The resulting hyperpolarization of the photoreceptor will cause a decrease in release of glutamate onto the downstream bipolar cells 8:45-9:55, signaling that light has entered the eye and contacted that photoreceptor. They will note in this video how the downstream response to a decrease in glutamate will differ depending on whether the bipolar cell is on or off 8:55-9:55.
“The Phototranduction Cascade” by Khan Academy Medicine (Ronald Sahyouni) is All Rights Reserved.
Visual Field Processing
The following video explains where light from locations of our visual field will act on the retinea 0:20-1:50. It then explores how signals generated by those lights will be integrated or segregated depending on what part of the retina they came from 1:51-4:09. The important structures to remember are the optic chiasm (where the optics nerves cross) 1:49-2:25 and the left and right LGN, which receive signals from the left side of each retina and right side of each retina respectfully 2:45-4:09.
“Visual Field Processing”by Khan Academy Medicine (Ronald Sahyouni) is All Rights Reserved.
Central Processing[4]
The myelinated axons of ganglion cells make up the optic nerves. Within the nerves, different axons carry different qualities of the visual signal. Some axons constitute the magnocellular (big cell) pathway, which carries information about form, movement, depth, and differences in brightness. Other axons constitute the parvocellular (small cell) pathway, which carries information on color and fine detail. Some visual information projects directly back into the brain, while other information crosses to the opposite side of the brain. This crossing of optical pathways produces the distinctive optic chiasm (Greek, for “crossing”) found at the base of the brain and allows us to coordinate information from both eyes.
Once in the brain, visual information is processed in several places, and its routes reflect the complexity and importance of visual information to humans and other animals. One route takes the signals to the thalamus, which serves as the routing station for all incoming sensory impulses except olfaction. In the thalamus, the magnocellular and parvocellular distinctions remain intact, and there are different layers of the thalamus dedicated to each. When visual signals leave the thalamus, they travel to the primary visual cortex at the rear of the brain. From the visual cortex, the visual signals travel in two directions. One stream that projects to the parietal lobe, the second stream projects to the temporal lobe.
Another important visual route is a pathway from the retina to the superior colliculus in the midbrain, where eye movements are coordinated and integrated with auditory information. Finally, there is the pathway from the retina to thesuprachiasmatic nucleus(SCN) of the hypothalamus. The SCN is a cluster of cells that is considered to be the body’s internal clock, which controls our circadian (day-long) cycle. The SCN sends information to the pineal gland, which is important in sleep/wake patterns and annual cycles.
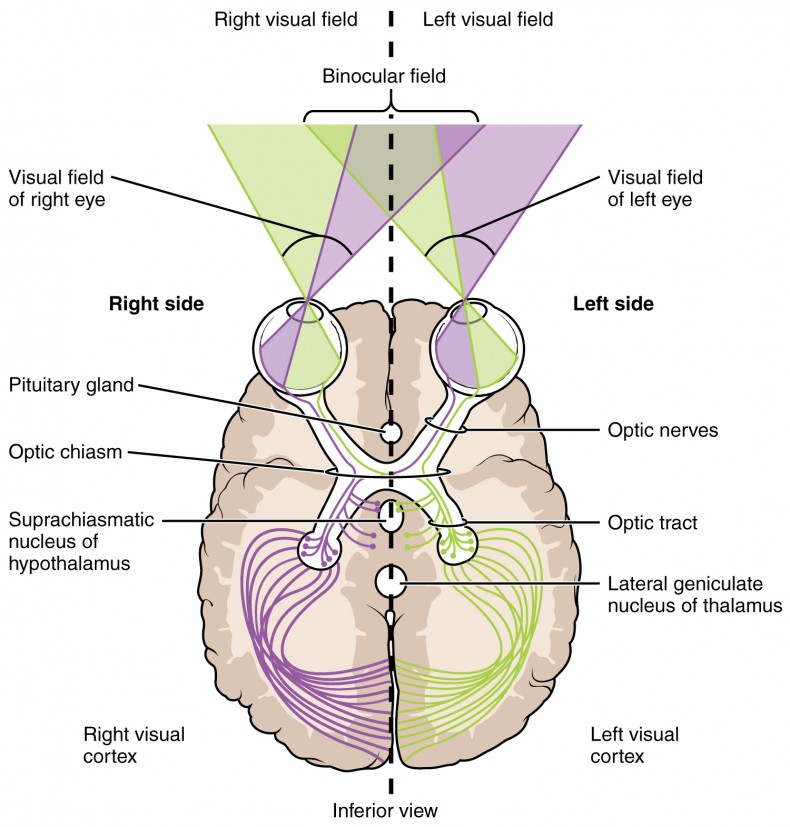
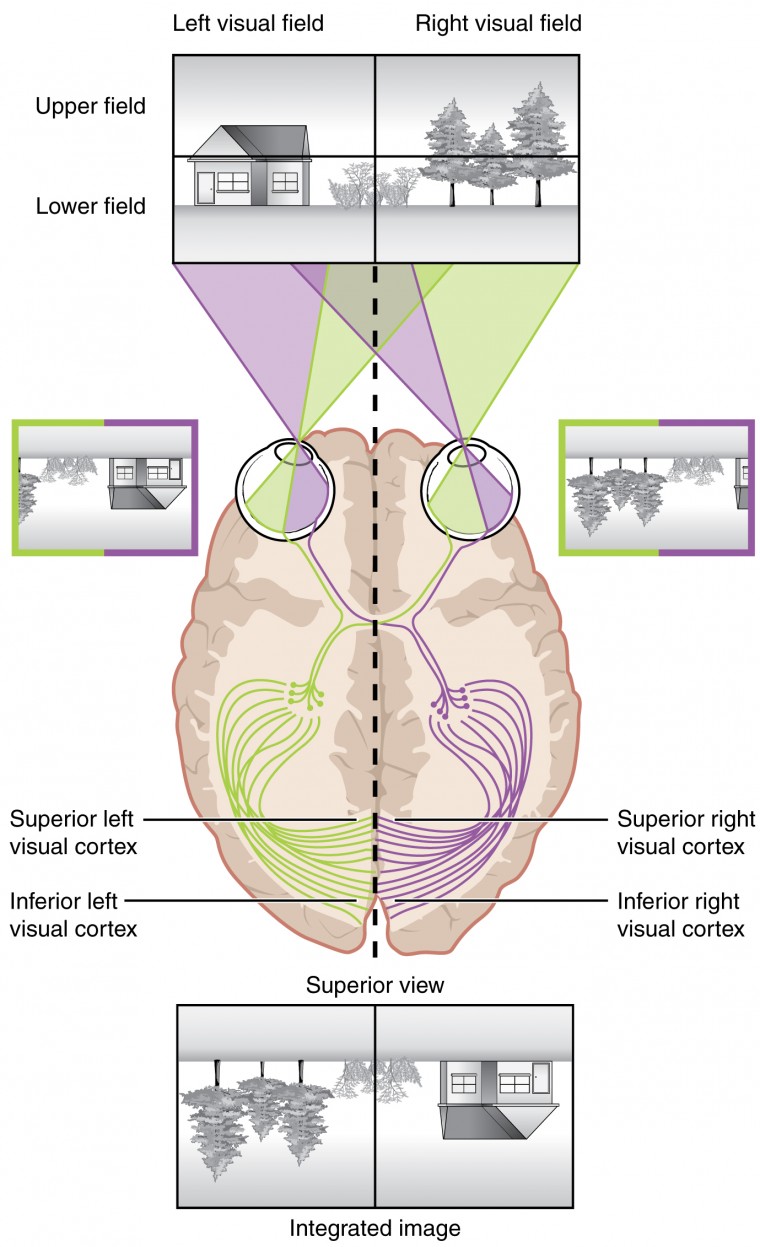
Tips From Past Students
Pick either the left or right visual field. Pick either the left or right eye. Practice either talking out or writing out the pathway that light would have to follow through the eye all the way until you reach image formation in the occipital lobe, with as much detail as possible. Make sure you’re comfortable with this.
The next step after that would be to identify exactly when information and segregated and integrated throughout that pathway.
Did you know that some individuals cannot recognize any faces? The inability to recognize people by their faces is a troublesome problem. It can be caused by trauma, or it may be inborn. Watch this video to learn more about a person who lost the ability to recognize faces as the result of an injury. She cannot recognize the faces of close family members or herself. What other information can a person suffering from prosopagnosia use to figure out whom they are seeing?
The video below describes the story of a woman who has had an injury to the area of the brain associated with the recognition of faces. This caused her to develop prosopagnosia which means she cannot recognize faces. Even the face of someone she is unfamiliar with cannot be recognized any better than the face of her own mother.
“Prosopagnosia” by Lasorlight Digital is All Rights Reserved.
The failures of sensory perception can be unusual and debilitating. Prosopagnosia, or face blindness, is a particular sensory deficit that inhibits an important social function of humans. The word comes from the Greek words prosopa, which means “faces,” and agnosia, which means “not knowing.”
Some people may feel that they cannot recognize people easily by their faces. However, a person with prosopagnosia cannot recognize the most recognizable people in their respective cultures. They would not recognize the face of a celebrity, an important historical figure, or even a family member like their mother. They may not even recognize their own face.
Prosopagnosia can be caused by trauma to the brain, or it can be present from birth. The exact cause of prosopagnosia and the reason that it happens to some people is unclear. A study of the brains of people born with the deficit found that a specific region of the brain, the anterior fusiform gyrus of the temporal lobe, is often underdeveloped. This region of the brain is concerned with the recognition of visual stimuli and its possible association with memories. Though the evidence is not yet definitive, this region is likely to be where facial recognition occurs.
Though this can be a devastating condition, people who suffer from it can get by—often by using other cues to recognize the people they see. Often, the sound of a person’s voice, or the presence of unique cues such as distinct facial features (a mole, for example) or hair colour can help the sufferer recognize a familiar person. In the video on prosopagnosia provided in this section, a woman is shown having trouble recognizing celebrities, family members, and herself. However, in some situations, she can use other cues to help her recognize faces.
Visual Field Processing and Lateral Geniculate Nucleus (LGN)[5]
The lateral geniculate nucleus (LGN) (also called the lateral geniculate body or lateral geniculate complex) is a relay center in the thalamus for the visual pathway. It receives a major sensory input from the retina. The LGN is the main central connection for the optic nerve to the occipital lobe. Each LGN has six layers of neurons (grey matter) alternating with optic fibers (white matter).
The LGN receives information directly from the retinal ganglion cells via the optic tract and from the reticular activating system. The LGN also receives strong feedback connections from the primary visual cortex. In humans as well as other mammals, the two strongest pathways linking the eye to the brain are those projecting to the dorsal part of the LGN in the thalamus, and to the superior colliculus.
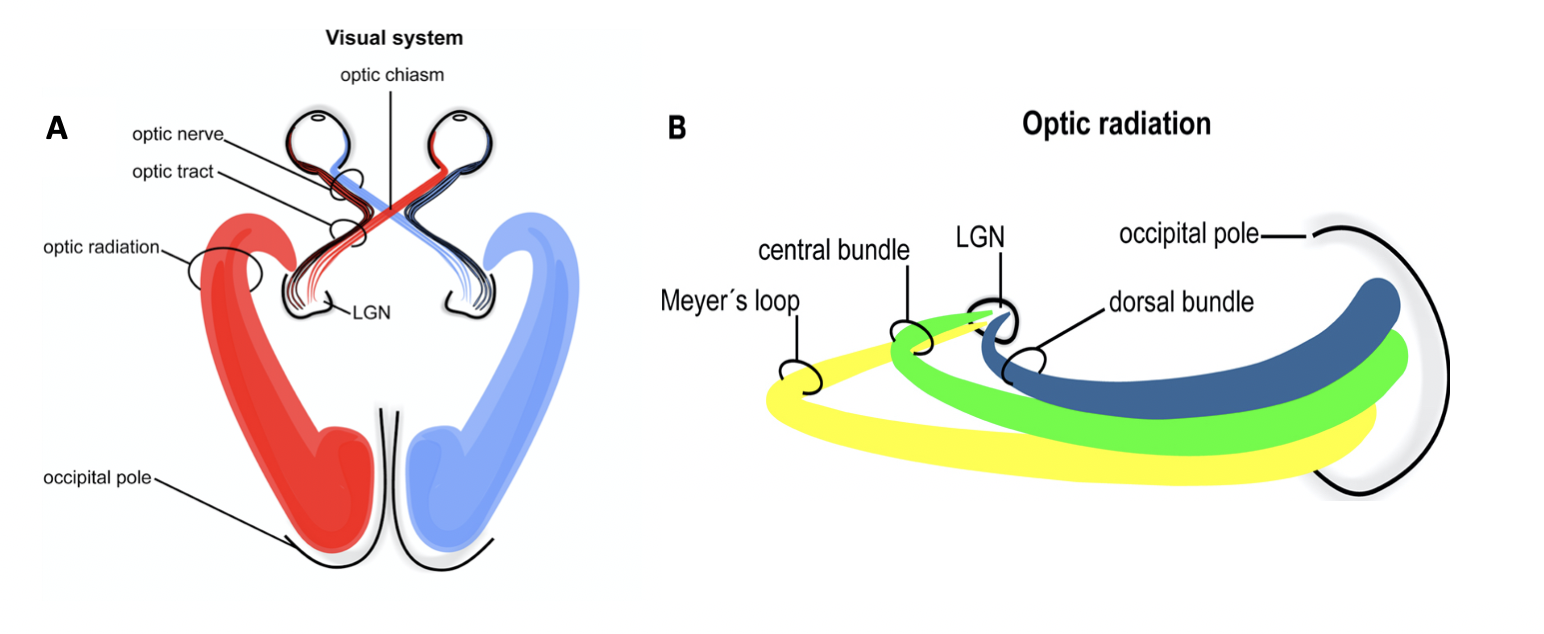
Inferior and Superior Colliculus
Neurons of the inferior colliculus project to the thalamus, which then sends auditory information to the cerebrum for the conscious perception of sound. The superior colliculus is the superior pair and combines sensory information about visual space, auditory space, and somatosensory space. Activity in the superior colliculus is related to orienting the eyes to a sound or touch stimulus. If you are walking along the sidewalk on campus and you hear chirping, the superior colliculus would coordinate the chirping with the awareness of the visual location of the tree right above you. That is the correlation of auditory and visual maps. If you suddenly feel something wet fall on your head, your superior colliculus integrates that with the auditory and visual maps and you know that the chirping bird just relieved itself on you. You want to look up to see the culprit but do not.
Ever wonder how the visual system can determine 3D perception using only a 2D image on the retina? Monocular depth cues are those that are the result of information within the two-dimensional visual field of one retina. One object that overlaps another object has to be in front. Relative size differences are also a cue. For example, if a basketball appears larger than the basket, then the basket must be further away. Based on experience, we can estimate how far away the basket is.
Binocular depth cues compare information represented in the two retinae because they do not see the visual field exactly the same. The centers of the two eyes are separated by a small distance, which is approximately 6 to 6.5 cm in most people. Because of this offset, visual stimuli do not fall on exactly the same spot on both retinae unless we are fixated directly on them and they fall on the fovea of each retina. All other objects in the visual field, either closer or farther away than the fixated object, will fall on different spots on the retina. The binocular disparity is the distance of an object away from the fixation point, which is the main tool we use for depth perception. This is the basis of 3-D movies, as they film with two side-by-side cameras simultaneously and then separate the images with 3-D glasses so each eye only sees one camera’s image.
When vision is fixed on an object in space, closer objects will fall on the lateral retina of each eye, and more distant objects will fall on the medial retina of either eye, as seen in figure 9. This is easily observed by holding a finger up in front of your face as you look at a more distant object. You will see two images of your finger that represent the two disparate images that are falling on either retina. These depth cues, both monocular and binocular, can be exploited to make the brain think there are three dimensions in two-dimensional information.
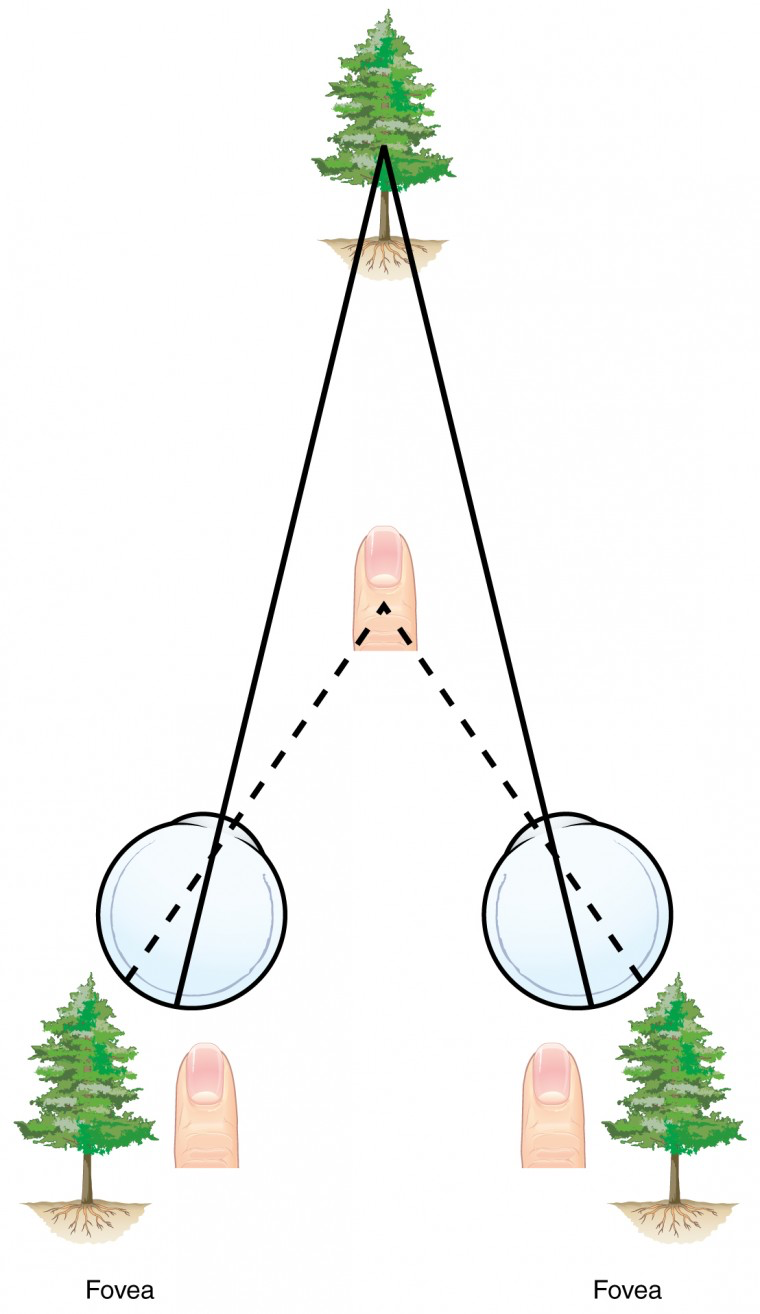
Visual Processing and the Brain
There are two main regions that surround the primary visual cortex in the occipital lobe that are usually referred to as areas V2 and V3 (the primary visual cortex is area V1). These surrounding areas are the visual association cortex. The visual association regions develop more complex visual perceptions by adding colour and motion information. The information processed in these areas is then sent to regions of the temporal and parietal lobes.
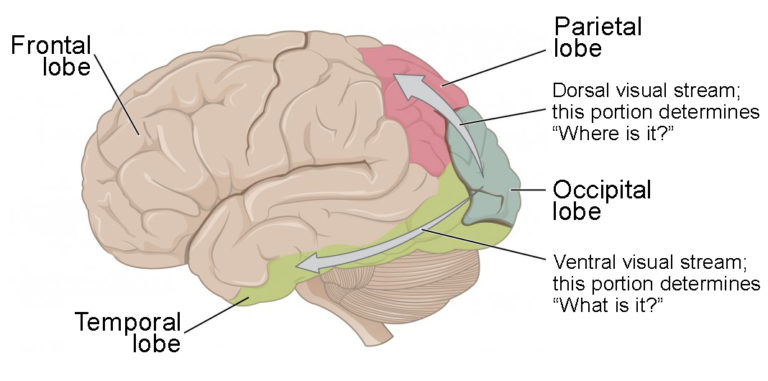
Visual processing has two separate streams from the occipital lobe: one into the temporal lobe and one into the parietal lobe. These are the ventral and dorsal streams, respectively, shown in figure 11.
The ventral stream identifies visual stimuli and their significance. Because the ventral stream uses temporal lobe structures, it begins to interact with the non-visual cortex and may be important in visual stimuli becoming part of memories.
The dorsal stream, also known as the what stream – is named so because incoming visual information will be sent to the temporal lobe to recognize what the object that we’re looking at is. Therefore, the end result of this stream is identifying (or recognizing) visual stimuli and their significance.
Testing Your Knowledge
Real-Life Scenario:
Let’s see how our knowledge of the two visual processing streams and their functions help us appreciate this seemingly simple real-life scenario. Consider walking into your lecture hall, spotting your friends and grabbing a seat next to them. At each stage in this process, explain which stream(s) (ventral or dorsal) would be used and why. Hint: The temporal lobe is what stores our memories while the parietal lobe tells us the relative location of our body in space.
The folding of the cortex maximizes the amount of gray matter in the cranial cavity. During embryonic development, as the telencephalon expands within the skull, the brain goes through a regular course of growth that results in everyone’s brain having a similar pattern of folds. The surface of the brain can be mapped on the basis of the locations of large gyri and sulci. Using these landmarks, the cortex can be separated into four major regions, or lobes, as seen in figure 11. The lateral sulcus that separates the temporal lobe from the other regions is one such landmark. Superior to the lateral sulcus are the parietal lobe and frontal lobe, which are separated from each other by the central sulcus. The posterior region of the cortex is the occipital lobe, which has no obvious anatomical border between it and the parietal or temporal lobes on the lateral surface of the brain. From the medial surface, an obvious landmark separating the parietal and occipital lobes is called the parieto-occipital sulcus. The fact that there is no obvious anatomical border between these lobes is consistent with the functions of these regions being interrelated.
Note: The occipital lobe is responsible for visual processing within the brain
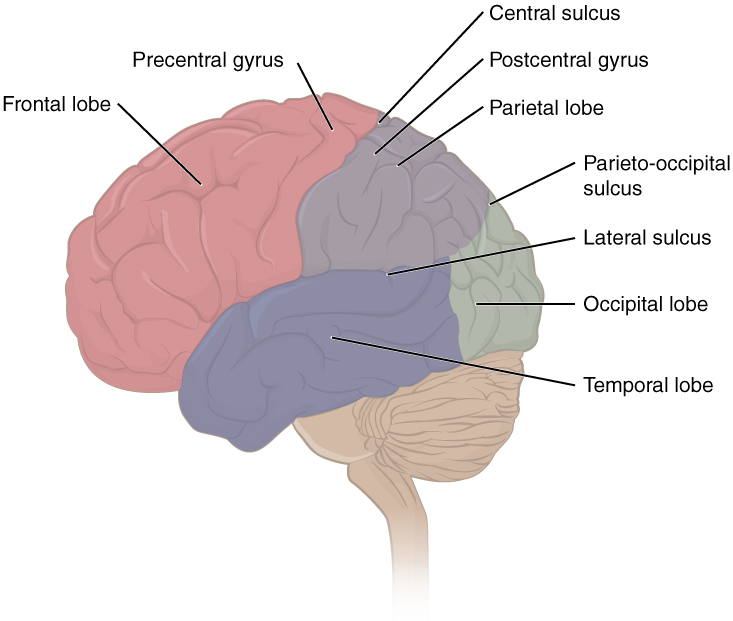
Tips From Past Students
Use this fill-in-the-blank activity to test your knowledge on the function of the optic chiasm, as well as the anatomy of the retina. This is a very useful tool that can be used to determine your level of understanding. The answers to the blanks can be found on pages 103 and 104 of the link attached above.
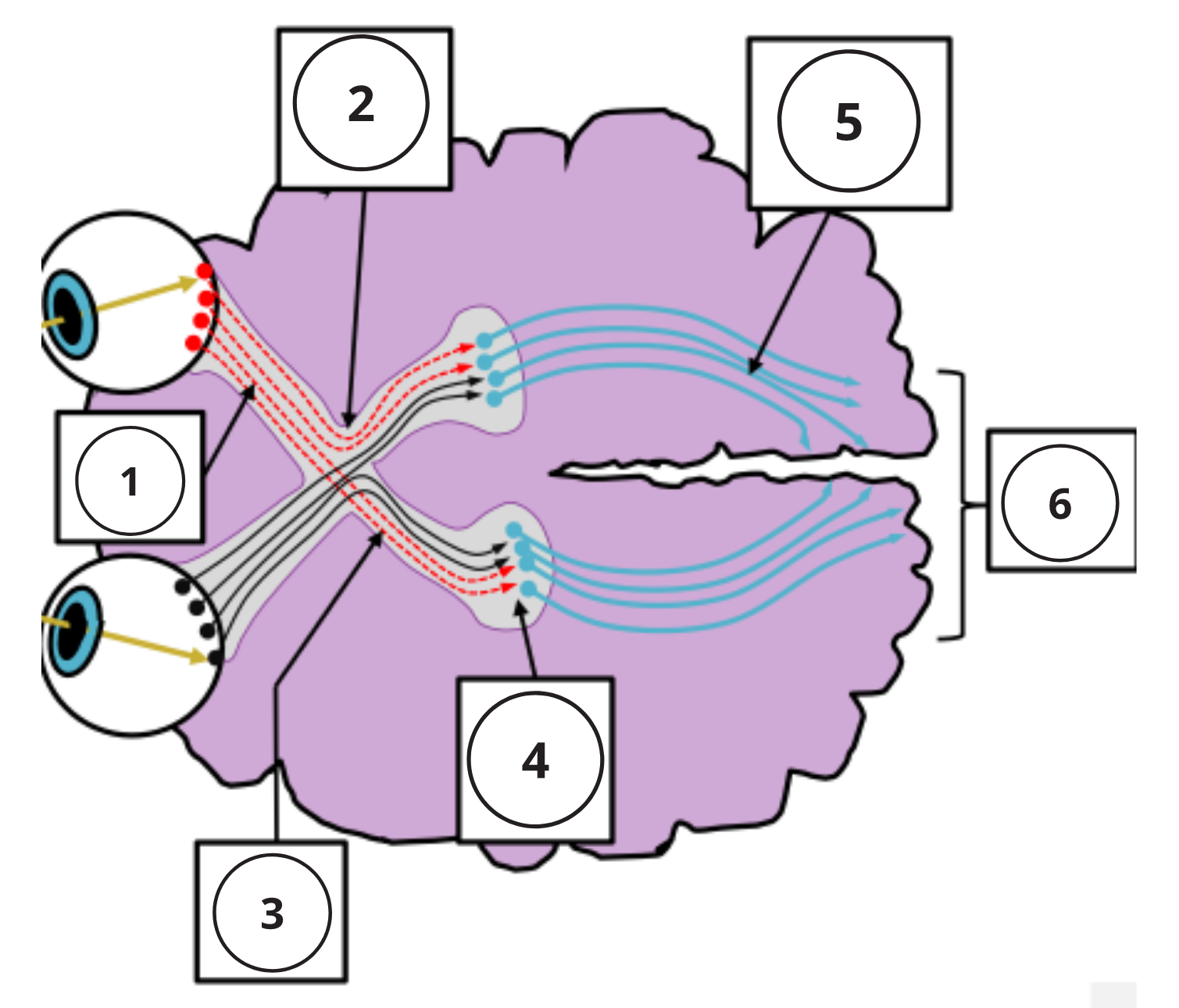
Key Takeaways
Consider the following concepts to help guide your studies:
- The anterior cavity of the eye contains the cornea, iris, pupil, lens, ciliary body and aqueous humor.
- The posterior cavity of the eye contains the neural tunic (retina), which has photoreceptors lining its most interior surface.
- There are two types of photoreceptors, rods and cones, which is the site of transduction of light.
- Rods are more sensitive to light, so they’ll be used more for seeing in dim environments.
- Cones are able to see colour and will produce a sharper image.
- When the eyes are fixated on an object the image will fall onto the fovea, the fovea contains only cones which is why the object fixated on is the sharpest.
- Bipolar cells, ganglion cells and neurons in the LGN all have photoreceptors that influence their activity in a centre-surround receptive field.
- When a photoreceptor is hit by a photon of light it will initiate a cascade of events that will hyperpolarize the photoreceptor.
- The activity of a visual pathway are often altered by adjacent pathways in a process called lateral inhibition.
- Signals travel through the optic nerve from the retina to the lateral geniculate nucleus (LGN) and then to the primary visual cortex (occipital lobe) with a pit stop in the superior colliculus to control eye movement.
- There are two streams of visual information that come from the occipital lobe and influence behavior, the ventral (what) pathway and dorsal (action, how) pathway.
- Landmarks to differentiate between lobes are the sulcus, which are folds that are considered borders between the lobes of the cortex.
Subchapter Quiz
The questions below can be used to assess your knowledge within this chapter. There are five multiple-choice questions that you should attempt without referring to your notes. The questions will provide you with responses to your answers to guide your studying but should not be used as your only resource.
Media Attributions
- Structure of Eye © OpenStax College is licensed under a CC BY (Attribution) license
- Human Eye Cross-Section © James Zagray is licensed under a CC BY (Attribution) license
- Photoreceptor © OpenStax College is licensed under a CC BY (Attribution) license
- Flag © OpenStax College is licensed under a CC BY (Attribution) license
- Receptive Fields © Wikipedia is licensed under a Public Domain license
- Cross-Sectional Bipolar Cell © Lindsay North is licensed under a CC0 (Creative Commons Zero) license
- Retinal Isomers © OpenStax College is licensed under a CC BY (Attribution) license
- Rhodopsin Activation © James Zagray is licensed under a CC BY (Attribution) license
- Segregation of Visual Field © OpenStax College is licensed under a CC BY (Attribution) license
- Topographic Mapping © OpenStax College is licensed under a CC BY (Attribution) license
- LGN Systems © Operative Neurosurgery is licensed under a CC BY-SA (Attribution ShareAlike) license
- Retinal Disparity © OpenStax College is licensed under a CC BY (Attribution) license
- Ventral and Dorsal Visual Streams © OpenStax College is licensed under a CC BY (Attribution) license
- Private: Lobes of the Cerebral Cortex © OpenStax College is licensed under a CC BY (Attribution) license
- Visual Information Pathway © Laird C. Sheldahl is licensed under a CC BY-SA (Attribution ShareAlike) license
- OpenStax College (n.d.). Vision. Retrieved April 10, 2021 from https://courses.lumenlearning.com/ap1/chapter/vision/ ↵
- OpenStax (n.d.) 39.5 Vision. Retrieved April 10, 2021 from https://cnx.org/contents/ZKf51Llg@1.166:fvwAeW4E@6/Vision ↵
- OER Commons (n.d.). Vision. Retrieved April 10, 2021 from https://www.oercommons.org/courseware/module/15125/overview ↵
- OpenStax College (n.d.). Central Processing. Retrieved April 10, 2021 from https://courses.lumenlearning.com/ap1/chapter/central-processing/ ↵
- Operative Neurosurgery (n.d.). Lateral Geniculate Nucleus. Retrieved April 10, 2021 from https://operativeneurosurgery.com/doku.php?id=lateral_geniculate_nucleus ↵
The innermost portion of the eye, also known simply as the retina. It lines the innermost part of the eye and contains photoreceptors.
Thin layer of tissue that lines the insides and back of the eye that acts to convert light that falls onto it into neural signals for visual transduction.
The transparent structure at the most anterior part of the eye. The cornea covers the entire anterior cavity of the eye and is the structure that refracts light the most.
A small, transparent structure deep to the pupil that will refract light onto the retina. The amount of refraction by the lens is modified by the ciliary muscles depending on the distance of the object.
The coloured part of the eye that is responsible for contracting or relaxing depending on the intensity of light in the environment. The iris is an involuntary sprinter muscle.
The part of the eye that generates aqueous humor and contains the ciliary muscles. Ciliary muscles will contract to bend the lens in order to appropriately focus the eye on an object at a certain distance away.
A transparent watery fluid that is in the anterior and posterior cavities of the eye. Since there is no blood supply to parts of the eye, such as the lens and cornea, the aqueous humor will provide nutrients.
Clear liquid found between the lens and the retina of the eyeball which helps maintain the shape of the eye and provide shock absorption.
A type of cell found in the retina that detects photons of light and converts them into electrical signals for the brain.
The first downstream retinal neuron from the photoreceptor. Assist in the process of transmitting the electrical signal from the photoreceptor to the RGC.
Also known as the outer plexiform layer, this is a layer of cells where synapsing of dendrites of horizontal cells, bipolar cells, and photoreceptor cells occurs in the retina.
The most proximal neuron in the retina of the visual pathway. Axons of these neurons make up the optic nerve.
Location where the synapsing of axons from bipolar cells occurs with dendrites of retinal ganglion cells.
Retinal neurons that are involved in the photoreceptor pathway, assisting with lateral inhibition.
Another name for the blind spot, where the retinal ganglion cells exit the eye as the optic nerve.
A cranial nerve of the brain that transfers visual information from the retina to the occipital lobe.
A small pit in the retina of the eye that is necessary for sharp central vision in actions such as reading. Light rays in this area fall directly on cone cells.
The sharpness of vision. The ability to discern patterns and shapes from a distance (measured using an eye chart).
The medial segment of the photoreceptor that contains the nucleus, mitochondria and ribosomes. Responsible for metabolism of the cell.
[of a photoreceptor] Modified cilia that are made up of discs filled with opsins that absorb light.
[cell type] Photoreceptor cells in the retina found on the outer edge of the eye that are highly sensitive to light and contribute to peripheral vision.
The less prevalent photoreceptor in the eye that allows us to see colour and creates a sharper image. The fovea of the eye has exclusively cones.
A G-Protein coupled receptor that is the most abundant protein in rod cells of the retina; plays a key role in low-light vision.
Light-sensitive proteins found in photoreceptors of the retina.
An excited neuron in one signal pathway inhibiting a neighbouring neuron of a different signal pathway.
[of vision] A theory that proposes that colour vision is controlled by three antagonistic colour pairings (black/white, red/green, blue/yellow) and when only one colour in the pair can be firing at a given time.
The retinal neurons that are responsible for carrying visual information from the photoreceptors into the brain. The axons of many ganglion cells constitute the optic nerve.
Large cells pathway that will signal to the brain about the form of an object, the depth of the object and/or the difference in intensity from multiple objects.
Small cells in the lateral geniculate nucleus that detect information regarding the colour and fine details of objects.
The crossing of the optic tract from the left eye that is heading to the right lateral geniculate nucleus with the optic tract from the right eye that is heading to the left lateral geniculate nucleus.
A region of the occipital lobe where information from both optic nerves converges; this region acts to sort and integrate visual information.
A region in the hypothalamus of the brain that resides just above the optic chiasm; regulates the body’s circadian rhythm.
Occurring on a twenty four hour cycle. The reason we feel like eating and sleeping at similar times of the day, even in the absence of light.
Midbrain nucleus mainly involved in auditory pathways. All auditory pathways travelling through the midbrain converge at the inferior colliculus.
A midbrain structure in the visual pathway that will adjust eye movements in response to the light stimuli it is receiving.
A pathway on the ventral side of the brain that carries visual information from the primary visual cortex to the temporal lobe; this pathway is responsible for object recognition and discrimination of forms of shapes.
The “action” or “where” or “how” pathway, which is the flow of visual information from the occipital lobe to the parietal lobe. The purpose of this pathway is to determine where in space the object in sight is and how the body must move to interact with that object.
A fold on the lateral side of the brain. Used as a landmark, or border, for where the frontal and parietal lobes end, posteriorly and laterally respectfully, and where the temporal lobe begins anterior-medially.
A fold on the dorsal side of the brain. Used as a landmark, or border, of the posterior edge of the frontal lobe and an anterior edge of the parietal lobe.
A deep groove at the posterior side of the brain that separates the posterior edge of the parietal lobe and the anterior edge of the occipital lobe.