Overcoming Space Between Cells
What happens when an action potential reaches the end of a neuron? How can the signal move from one neuron to the next? This subchapter will introduce the physiological procedures that enable intercellular communication through the formation of various types of synapses.
Learning Outcomes
In this section you will learn…
- Define and explain synaptic transmission.
- Describe the differences between Electrical and Chemical synapses, including ionotropic and metabotropic chemical synapses.
- Differentiate between nerve-skeletal muscle and nerve-nerve connections.
- Describe EPSPs and IPSPs and the different types of summation.
- Explain the process of Long-Term Potentiation.
Synaptic Transmission
The synapse or “gap” between neurons is the extracellular space where information is transmitted from one neuron to another. Sometimes, this space is also called the synaptic cleft. The neuron transmitting the signal is called the presynaptic neuron, and the neuron receiving the signal is called the postsynaptic neuron.
Tips from Past Students
The terms “presynaptic” and “postsynaptic” are relative. Often, a signal is propagated across more than one neuron, making most neurons both presynaptic and postsynaptic. For example, in the above diagram, Neuron 1 is transmitting a signal to Neuron 2, while Neuron 2 is transmitting a signal to Neuron 3. Since Neuron 2 is both receiving a signal and transmitting it to the next neuron, it would be considered postsynaptic relative to Neuron 1 and presynaptic relative to Neuron 3.
There are two types of synapses: electrical synapses and chemical synapses. The distinction has to do with how the signal (action potential) moves from one cell to another. This signal transmission can happen electrically, in which case we would have an electrical synapse, or chemically, in which case we would have a chemical synapse.
Electrical Synapse
In an electrical synapse, the presynaptic and postsynaptic membranes are physically connected by channel proteins forming gap junctions. Gap junctions allow current to pass directly from one cell to the next. So, the action potentials from the first neuron continue flowing down the channel proteins to the second neuron. Signaling in electrical synapses is essentially instantaneous, making this type of synapse very important for some key reflexes. This is because there is no conversion of energy from one form to another. We had an action potential and we still have an action potential. The electrical signal didn’t get converted to something else to be able to overcome the space between the neurons.
Electrical synapses may also propagate signals bidirectionally, meaning the signal can propagate in either direction. This is again because there is no conversion of energy from one form to another. If the action potential moved from neuron A to neuron B, then structurally, there is nothing stopping it from moving from neuron B back to neuron A. Under normal conditions, this doesn’t happen because of refractory periods. While electrical synapses are not prevalent in the nervous system, they are essential to the heart and smooth muscle of the vasculature.
Test Your Knowledge
Thinking Beyond:
The refractory period prevents the action potential from traveling backward. What do you suspect would happen if an action potential did travel backward? Hint: How would this affect the unidirectional flow of information, and more importantly, the overall goal of the action potential (i.e., why the action potential was sent, and what it is seeking to accomplish)?
Chemical Synapse
In a chemical synapse, a neurotransmitter is released from the presynaptic cell and diffuses across the synaptic cleft to the postsynaptic cell. So, here we see a conversion of electrical energy (action potential in neuron A) to chemical energy (the release of neurotransmitter). Because chemical synapses depend on the release of neurotransmitters, signal propagation is not instantaneous. Signaling is also unidirectional, meaning the signal can only propagate in one direction. This is because to go backward, we would need to convert the action potential to a chemical signal first, and due to asymmetry, this isn’t possible (there is no machinery to enable the cell to do that). The machinery that releases the neurotransmitter is found at the end of the axon, not on the cell body dendrites. The receptors that accept the neurotransmitter are found on the dendrites, not at the end of the axon. Chemical synapses are prevalent in the nervous system and are also found in nerve-skeletal and nerve-smooth muscle connections.
There are two types of chemical synapses. A chemical synapse is considered ionotropic if the post-synaptic cell opens an ion channel in response to the neurotransmitter binding to its receptors. If the post-synaptic cell changes metabolic activity in response to the neurotransmitter binding to its receptors, then it is a metabotropic chemical synapse. These types are explained in the diagram below.
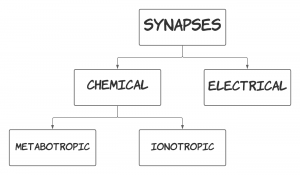
Ionotropic Chemical Synapse
Ionotropic receptors are complexes made up of 4 to 5 proteins called subunits. There are different kinds of subunits that can come together to form a receptor and a pore or a receptor and a channel in the centre of the subunits. So, the receptor is simultaneously a receptor (something that binds a ligand) and also a channel/pore (something that lets things through). The types of subunits that make up the receptor determine what ion that channel is selective for. For example, some channels are selective for sodium ions while other channels are selective for potassium ions. At rest, when no neurotransmitter is bound to the receptor, the channel is closed and will not allow any ions through.
A neurotransmitter selective for a channel will have a orthosteric binding site where it will bind and cause the channel to open and allow ions to flow in or out of the cell. The neurotransmitter itself doesn’t actually go into the cell. It only acts as a key to open the channel, which will then allow certain ions to enter. Those ions are already present in the surrounding environment. Their direction of entry is governed by their equilibrium potential and other principles we talked about in the bioelectricity section. As soon as the neurotransmitter dissociates from the receptor, the channel quickly closes. The binding of the neurotransmitter to the receptor causes a very rapid change in the post-synaptic cell and once the ligand (neurotransmitter) dissociates, the response terminates rapidly as well. Because ionotropic receptors require a ligand (neurotransmitter) to open them and they allow ions to flow through, they are also referred to as ligand-gated ion channels.
Remember, when referring to an “ionotropic synapse” these are the channels that are present on the post-synaptic cell. We will now discuss two examples of ionotropic synapses.
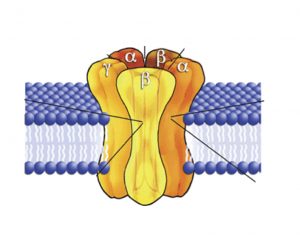
Example 1: The Neuromuscular Junction
- An action potential (AP) travels down the presynaptic axon.
- Once the AP reaches the axon terminal, voltage-gated calcium (Ca2+) channels in the presynaptic membrane open. Ca2+ enters the presynaptic terminal, resulting in an increase in intracellular Ca2+ concentration.
- Ca2+ ions associate with the proteins on the outer surface of neurotransmitter vesicles, promoting the fusion of the neurotransmitter-containing vesicles to the presynaptic membrane. In the neuromuscular junction, the neurotransmitter is acetylcholine.
- Acetylcholine is released from its vesicles into the synaptic cleft. From here, acetylcholine will either be degraded by enzymes, taken up again and recycled by the pre-synaptic neuron, or it will bind to ligand-gated sodium (Na+) channels on the postsynaptic membrane, causing them to open. For the rest of this example, we will focus on acetylcholine binding to these ligand-gated channels.
- Once acetylcholine binds to the ligand-gated channels, those channels will open, letting Na+ into the cell and depolarizing it.
- Local current moves the depolarization to an area with voltage-gated Na+ and potassium (K+) channels.
- These channels respond to depolarization.
- An AP is initiated in the post-synaptic cell.
Test Your Knowledge
Thinking Beyond:
We have learned that when neurotransmitters are released across a neuromuscular junction, they will either be degraded, reabsorbed or they will bind to the post-synaptic membrane. There is a small portion of neurotransmitters that diffuse away before being reabsorbed or degraded. This is called volume transmission. Where do you think these neurotransmitters go? Would this have a significant effect? Hint: Where else could they bind? Is there enough of them to create a response?
This video reviews the steps of synaptic transmission at the neuromuscular junction. Specifically, this helps to clarify how the signal (AP) triggers a response, the axon terminal and muscle fibre connection, and acetylcholine transmission across the synaptic cleft. Don’t worry about the content past 2:30.
Tips from Past Students
Remember, if there is an AP in the presynaptic terminal that causes the release of acetylcholine, an AP in the skeletal muscle will always follow. You may have previously learned about muscle contraction and the Sliding Filament Theory. Keep in mind that actin and myosin can only interact after an AP is sent down the muscle cell membrane and reaches a T-tubule where that process begins. The neuromuscular junction has to release acetylcholine for the muscle to contract.
Ever wonder how Botox works? Botulinum Toxin (AKA Botox) acts at the neuromuscular junction to prevent the docking of the synaptic vesicles that are filled with acetylcholine. This prevents exocytosis of acetylcholine into the synaptic cleft and doesn’t allow muscle contraction to occur. The end result is paralysis of the muscle!
Example 2: Nerve-Nerve Connection
The first 3 steps (the presynaptic activity) are the same between the two different types of chemical synapses
-
- An action potential (AP) travels down the presynaptic axon
- Once the AP reaches the axon terminal, voltage gated Ca2+ channels in the presynaptic membrane open. Ca2+ enters the presynaptic terminal, resulting in an increase in intracellular Ca2+ concentration.
- Ca2+ ions associate with the proteins on the outer surface of neurotransmitter vesicles that are stored in the terminal, promoting the fusion of the neurotransmitter vesicles to the presynaptic membrane. Unlike the neuromuscular junction, the neurotransmitter is not restricted to acetylcholine.
- Neurotransmitter is released into the synaptic cleft
- Neurotransmitter bind to ligand-gated ion channels on the post-synaptic cell
- Post-synaptic membrane changes the membrane potential
Whether the post-synaptic cell depolarizes or hyperpolarizes depends on the type of ionotropic receptor present on that membrane. If the ligand binds to a Na+ or Ca2+ channel, the cell depolarizes, making the membrane more positive which causes an excitatory post-synaptic potential (EPSP). This makes sense because we know there is more Na+ and Ca2+ on the outside of the cell, so if the ligand causes one of these two channels to open Na+ and Ca2+ will flood into the cell. Since they are both positively charged molecules, the resting membrane potential increases (becomes less negative) and gets closer to the firing threshold. On the other hand, if the ligand binds to a K+ or chloride (Cl–) channel, the cell hyperpolarizes, making the membrane more negative which causes an inhibitory post-synaptic potential (IPSP). Thinking through this again, there are more K+ inside the cell, so when its ion channels open, those ions rush out of the cell. Cl– however, is more prominent outside of the cell, so similar to Na+ and Ca2+, when a Cl– channel opens Cl– influxes causing the membrane potential to become more negative. So, which receptor is bound is what determines whether the neuron will experience an EPSP or an IPSP. Which receptor will get bound by the ligand/neurotransmitter depends on the ligand/neurotransmitter. In neuromuscular junctions, the ligand is always going to be acetylcholine, whereas, in nerves, there are multiple possible ligands that can get released. If a neurotransmitter (the ligand in this case) targets a ligand-gated Cl- channel, then we can predict that the neuron will experience an EPSP from this interaction.
Tips from Past Students
If you’re struggling to remember which ions are more concentrated inside vs outside the cell at rest here is my little trick. All of them have higher concentrations outside of the cell except for K+. Because this is a Human KINetics course, there is more K+ IN the cell. Quirky, I know.
Communication Between Neurons[1]
All types of graded potentials will result in small changes of either depolarization or hyperpolarization in the voltage of a membrane. These changes can lead to the neuron reaching threshold if the changes add together, or summate. The combined effects of different types of graded potentials are illustrated in figure 3. If the total change in voltage in the membrane is a positive 15 mV, meaning that the membrane depolarizes from −70 mV to −55 mV, then the graded potentials will result in the membrane reaching threshold.
For receptor potentials, threshold is not a factor because the change in membrane potential for receptor cells directly causes neurotransmitter release. However, generator potentials can initiate action potentials in the sensory neuron axon, and postsynaptic potentials can initiate an action potential in the axon of other neurons. Graded potentials summate at a specific location at the beginning of the axon to initiate the action potential, namely the initial segment. For sensory neurons, which do not have a cell body between the dendrites and the axon, the initial segment is directly adjacent to the dendritic endings. For all other neurons, the axon hillock is essentially the initial segment of the axon, and it is where summation takes place. These locations have a high density of voltage-gated Na+ channels that initiate the depolarizing phase of the action potential.
The summation can be spatial or temporal, meaning it can be the result of multiple graded potentials at different locations on the neuron, or all at the same place but separated in time. Spatial summation is related to associating the activity of multiple inputs to a neuron with each other. Temporal summation is the relationship of multiple action potentials from a single cell resulting in a significant change in the membrane potential. The spatial and temporal summation can act together, as well.
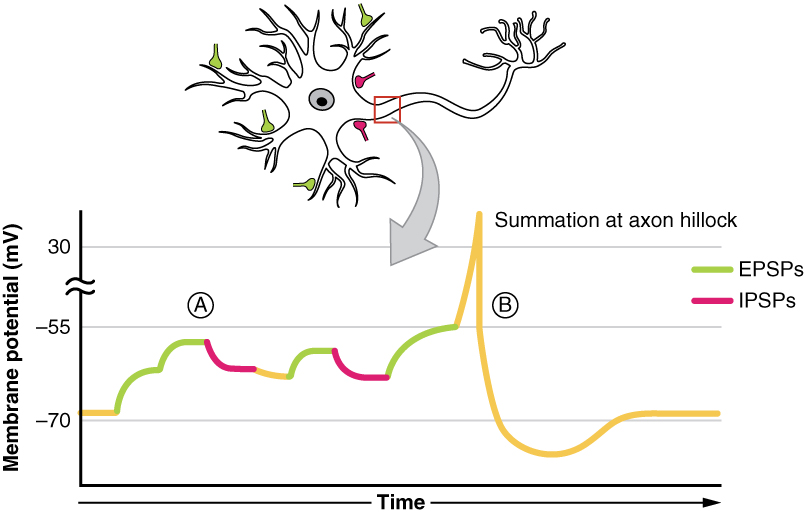
Tips from Past Students
Note that figure 3 is touching on one of the biggest themes of this unit: integration. The principle of summation is just one of the methods of integration in the body, so be sure to look over the other methods!
EPSPs and IPSPs
Post-synaptic potentials (PSP) are the change in membrane potential of the postsynaptic dendrite. An excitatory post-synaptic potential (EPSP) occurs when the post-synaptic membrane potential moves closer to threshold (depolarizes), facilitating the generation of an AP. An inhibitory post-synaptic potential (IPSP) occurs when the post-synaptic membrane moves further away from threshold (hyperpolarizes), inhibiting the generation of an AP.
Assuming the voltage-gated Na+ channel has a threshold of −65 mV and the neuron’s resting membrane potential is −70 mV, then one EPSP cannot generate an AP on the post-synaptic membrane. This is because one EPSP causes a +2mV change in the membrane potential, which is not sufficient to get the membrane potential to threshold. Therefore, EPSPs must summate in order to cause an AP. There are two ways summation can happen. Spatial summation occurs when there are multiple inputs from different pre-synaptic neurons arriving at the post-synaptic neuron at the same time. If there are enough EPSPs at the same time, the membrane can reach threshold and an AP can be initiated. Temporal summation occurs when there is multiple inputs from the same pre-synaptic neuron arriving at the post-synaptic neuron in rapid succession.
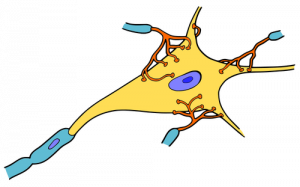
You can also think of it as integration, in the sense that if multiple depolarizations occur at the same time, they add up to a larger depolarization. Similarly, multiple hyperpolarizations occurring at the same time will add together to produce a larger inhibitory potential. In figure 4 you can see there are multiple terminal buttons (red finger-like things) from other neurons synapsing onto the cell body of the yellow neuron. Each of those terminal buttons conducts a potential (depolarization or hyperpolarization) onto the yellow neuron. Once all of those potentials reach the axon hillock (where the yellow and blue meet) they summate, so if they add up to −50mV an action potential will fire down the axon. An EPSP will increase or make the membrane potential less negative (closer to threshold), whereas an IPSP will make it more negative (further from threshold). Whether we have an EPSP or an IPSP will depend on the channel that was activated. Whether or not an action potential will happen depends on the sum of all of the received EPSPs and IPSPs because an action potential is an all or nothing event – it either happens or it doesn’t.
This video goes into a further explanation of summation. The first 2:50 reviews what summation is and how it relates to the firing of an AP. If you are comfortable with this concept, the two types of summation are discussed and illustrated after 2:50.
Tips from Past Students
The easiest way to keep the two types of summation straight is to remember Spatial summation occurs in Space, whereas Temporal summation occurs in Time!
Metabotropic Chemical Synapse
Similar to ionotropic synapses, metabotropic synapses require a non-lipid soluble substance (neurotransmitter or hormone) to bind to the receptor. This is because a lipid-soluble substance will just diffuse across the hydrophobic cell membrane instead of binding to receptors on the cell membrane. The chart in your notes breaks down the different types of receptors and their associated non-lipid soluble substances. For example, epinephrine (AKA adrenaline) and norepinephrine (AKA noradrenaline) both bind to adrenergic receptors. There are two different types of adrenergic receptors, α, and β, which are both metabotropic receptors. While norepinephrine and epinephrine can both bind to both α and β adrenergic receptors, epinephrine binds to β with a slightly greater affinity than norepinephrine. Conversely, norepinephrine binds the α-adrenergic receptors with a slightly greater affinity than epinephrine. If a neurotransmitter binds an α-adrenergic receptor, the end result for the muscle is vasoconstriction, whereas binding to a β-adrenergic receptor will lead to vasodilation. You will see the chain of events leading to this end result as you move through this section. This should help clear up any confusion regarding the chart about neurotransmitters and receptors! Think about the pharmacological implications of this. What would happen if someone took a chemical that blocked alpha receptors in their blood vessels? What condition might this help mitigate?
Let’s look at the structure of metabotropic receptors! The receptor weaves through the membrane creating 7 transmembrane domains and does not have a pore-like ionotropic receptors do. If they don’t have a pore, how do they work? They possess a G-protein on the intracellular side of the membrane that is associated with the 7th transmembrane domain. As depicted in figure 5, a G-protein is inactive when a GDP molecule is bound. When the non-lipid soluble substance interacts with the metabotropic receptor, it causes the receptor to interact with the G-protein which removes the GDP and replaces it with GTP, activating it (figure 3). Now the G-protein can cause an ion channel to open or close, or it can interact with an effector enzyme to change the levels of second messenger in the cell. These receptors tend to act slower than ionotropic receptors but can have longer lasting effects.
Again, this is what is the receptor looks like on the post-synaptic cell when someone says it is a “metabotropic synapse”. It could also be referred to as a “G-protein coupled receptor”.
Figures 5, 6 and 7 show a metabotropic receptor embedded into the membrane of a cell. Each time the receptor crosses through the membrane is considered a transmembrane domain, of which there are 7. These figures are at course level, however, some of the detail displayed is above course level. You do not need to know that there are 3 subunits in a G-protein and that GDP/GTP is bound specifically to the alpha subunit. It is also for your interest that the subunit breaks apart when GTP binds into an α-ATP dimer and a β-γ dimer. The purpose is that you get an understanding of the structural and functional differences between ionotropic and metabotropic receptors.
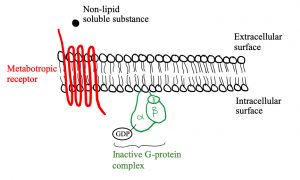
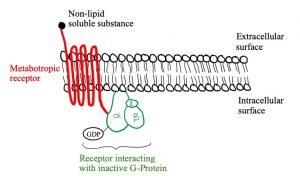
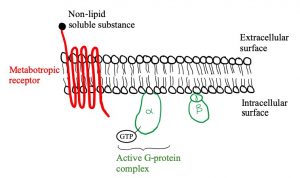
ATP is converted to cAMP by adenylate cyclase if adenylate cyclase is stimulated by a G-protein. In this case, adenylate cyclase is the “effector enzyme” referred to earlier (remember “ase” indicates an enzyme). Similarly, guanylate cyclase converts GTP to cGMP. A third effector enzyme is phospholipase C, which converts PIP2 to either diacylglycerol (DAG) or inositol triphosphate (IP3). cAMP, cGMP, DAG and IP3 are four out of the five second messengers in the human body. The fifth is Ca2+, which is involved in many different aspects of the body.
Okay, now that we’ve made a second messenger, what’s next? One way these second messengers can work is by activating protein kinases. A kinase is an enzyme that phosphorylates proteins. There are specific protein kinases associated with each second messenger system, cAMP is associated with PKA, which means cAMP activates PKA. cGMP is associated with PKG, which means that cGMP activates PKG. DAG and IP3 are associated with PKC, which means they activate PKC. Once active, PKA, PKG, and PKC can then go ahead and phosphorylate substrates. Ca2+ has an associated protein kinase (calcium-calmodulin kinase II), that is beyond this course, however, you may run into it if you are taking psychology classes so just be aware it does exist! Protein kinases are enzymes that ADD a phosphate onto a protein, which is one way to activate the protein. The kinase removes the phosphate from ATP, converting it to ADP, and adds the removed phosphate to the protein. The activation of different proteins is what then leads to a change in cellular effects.
One example you’re given is norepinephrine binding to an α-adrenergic receptor. When myosin is phosphorylated by myosin light-chain kinase (MLCK), myosin then binds to actin which causes a smooth muscle contraction. Myosin and actin-binding, and the muscle contraction is the change in cellular function referred to earlier.
Phosphatases do the opposite of protein kinases and “turn off” a protein by removing a phosphate.
The diagram below depicts the steps involved in a metabotropic chemical synapse. A ligand binds to a metabotropic receptor causing the G-protein to dissociate. The now active G-protein can cause an ion channel to open or close, or it can interact with an effector enzyme: adenylate cyclase, granulate cyclase or phosphorylase C. These enzymes change the levels of the second messengers shown: ATP, GTP or PIP2.
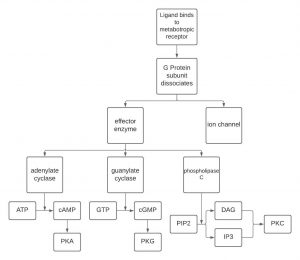
Let’s think about this in an applied sense. Would you rather have α or β adrenergic receptors on the vasculature of your heart? Think about it, if you had α-adrenergic receptors there would be vasoconstriction, which you definitely don’t want to constrict the vessels delivering blood to your heart! That being said, there are only β-adrenergic receptors on the blood vessels to the heart. This comes back in HK*3810 during the heart and vasculature unit.
Now think about pulmonary circulation (carrying deoxygenated blood to the lungs from the heart and then oxygenated blood back to the heart from the lungs). You don’t really want to mess with this blood flow at all. In order to maintain blood flow, these vessels are equally decorated with α- and β-adrenergic receptors, so there is a balance between vasoconstriction and vasodilation.
Ever wonder how Viagra works? Viagra (AKA sildenafil) is a PDE5 inhibitor, meaning that it prevents the inactivation of cGMP. By taking Viagra, cGMP cannot be inactivated which increases the levels and prolongs the action of cGMP in the penile smooth muscles, relaxing the smooth muscle, increasing blood flow and producing an erection.
Long-Term Potentiation
How do we learn? This slide goes through the detailed process of how we learn since you’re not making new nerves in your brain every time you learn something new (they wouldn’t all fit)! What actually happens is the signal between 2 neurons is strengthened which is referred to as Long Term Potentiation. Glutamate is the neurotransmitter that is involved and has 3 receptors it can bind to, AMPA, NMDA and a metabotropic receptor. Here’s what happens:
An AP comes down the pre-synaptic neuron, causing the opening of voltage-gated Ca2+ channels. Calcium floods into the cell and promotes the fusion of vesicles containing Glutamate to the pre-synaptic cell membrane. Glutamate is released into the synapse.
Let’s start with the metabotropic receptor. We know metabotropic receptors are G-protein coupled, so when Glutamate binds to this receptor there is an intracellular cascade causing PIP2 to be broken down to IP3 or DAG (we know these are both second messengers). These second messengers then help the Endoplasmic Reticulum to release Ca2+ from its stores. This is one of two places that causes an increase in intracellular calcium.
Glutamate released into the synapse from the pre-synaptic cell also binds to AMPA and NMDA receptors. The AMPA receptor is a ligand-gated Na+ channel. The opening of the AMPA receptor produces a small EPSP (2 mV) because of a small influx of Na+ ions. The NMDA receptor is a ligand-gated Ca2+ channel (our second source of increased intracellular Ca2+). This NMDA receptor has a Mg2+ plug that needs 60mV of depolarization in order to release the magnesium and open. So how would we get a greater EPSP to release the Mg2+ plug? By increasing the rate of firing of AP (AKA temporal summation) on the pre-synaptic cell, there is an increased amount of glutamate released into the synapse that can bind to the AMPA receptors, causing a greater influx of Na+, thus, causing more EPSPs that will summate to eventually depolarize the membrane potential to 60mV. Once this threshold is reached, Mg2+ will come off and Ca2+ will be able to enter through the NMDA receptor channel.
Tips from Past Students
Remember in biomechanics we talked about tetanus of a muscle being caused by APs being rapidly fired, summating and causing sustained muscle contraction because of the constant stimulation? This is kind of the same thing in the sense that APs being fired rapidly causes prolonged activation of a receptor!
Now that both the AMPA and NMDA ion channels are open, there is an influx of Na+ (from AMPA) and Ca2+ (from NMDA). Now it’s time for Ca2+ to get involved. Ca2+ has 2 roles in this process which include an increase in the number of AMPA receptors on the post-synaptic membrane, which makes the post-synaptic cell more sensitive to Glutamate and the stimulation Nitric Oxide Synthase (NOS) to convert Arginine into Nitric Oxide (NO).
NO is lipid soluble so it can easily pass through the membrane of the post-synaptic cell. Its role at the pre-synaptic cell is to increase the amount of Glutamate released during each AP. Both of these effects of calcium increase the connection between the pre-synaptic and post-synaptic cells. This is how we learn!
This is a great video to review the effects of Glutamate and the process of Long Term Potentiation! It goes over the ion movement and receptor activation as well as the effects of Ca2+ on enhancing the synapse. It does discuss a specific protein that calcium activates (CAM and CaKMII) that you do not need to know.
Key Takeaways
Consider the following concepts to help guide your studies:
- A synapse describes the process of 2 neurons communicating over the space between them (can be electrical or chemical).
- Electrical synapses conduct through gap junctions which are protein channels connecting the presynaptic and postsynaptic neurons.
- Chemical synapses convert the electrical signal from an AP to a neurotransmitter in order to cross the synaptic cleft and communicate with the postsynaptic neuron.
- Chemical synapses are ionotropic if the postsynaptic cell opens an ion channel in response to a neurotransmitter and are metabotropic if the postsynaptic cell releases a G-protein to initiate a biochemical cascade.
- The Neuromuscular Junction connects nerves to muscles using an ionotropic synapse with acetylcholine as the only neurotransmitter.
- Nerve-Nerve synapses are also generally chemical synapses, however, aren’t restricted to only acetylcholine.
- Synapses can cause either excitation or inhibition in the postsynaptic neuron which is dependent on the type of ion channels that get opened. Excitatory postsynaptic potentials (EPSPs) depolarize the membrane, bringing it closer to threshold, whereas inhibitory postsynaptic potentials (IPSPs) hyperpolarize the membrane bringing it further away from threshold.
- In isolation, EPSPs and IPSPs don’t have a large effect on the postsynaptic neuron. Their effects are added in the process of summation, which is either temporal (many inputs from the same neuron quickly) or spatial (inputs from multiple neurons at the same time).
Subchapter Quiz
The questions below can be used to assess your knowledge within this chapter. There are five multiple-choice questions that you should attempt without referring to your notes. The questions will provide you with responses to your answers to guide your studying but should not be used as your only resource.
Media Attributions
- Neurons © Ashley Fisher is licensed under a All Rights Reserved license
- Private: Synapses-4 © Ghaid Asfour is licensed under a All Rights Reserved license
- Ionotropic Receptor © David M. Lovinger is licensed under a CC BY-SA (Attribution ShareAlike) license
- Postsynaptic Potential Summation © OpenStax College is licensed under a CC BY (Attribution) license
- neuron © Open Clipart is licensed under a Public Domain license
- Metabotropic Receptor © Jordyn Dickson is licensed under a All Rights Reserved license
- Rc G-protein interaction © Jordyn Dickson is licensed under a All Rights Reserved license
- Active G-protein © Jordyn Dickson is licensed under a All Rights Reserved license
- Private: synapses © Ghaid Asfour is licensed under a All Rights Reserved license
- https://opentextbc.ca/anatomyandphysiology/chapter/12-5-communication-between-neurons ↵
Space between two cells, consisting of the pre-synaptic neuron, synaptic cleft, and the post-synaptic neuron. Involved in the transmission of information between two cells.
Small gap between the pre-synaptic and post-synaptic neuron, about 20nm wide.
Neuron at the synapse that transmits the signal to the post-synaptic neuron.
Neuron at the synapse that receives the signal from the post-synaptic cell.
Intracellular connection that connect the cytoplasm of two cells that allow molecules and ions to pass through.
Fast acting receptor that has an ion channel at its centre that is controlled by the binding of neurotransmitters to specific sites on the receptor. AKA ligand-gated ion channel.
Binding site on a receptor where the endogenous ligand binds. AKA the active site.
Depolarization that causes the membrane potential to move closer to firing threshold.
Hyperpolarization of the post-synaptic cell that causes the membrane to move further from firing threshold.
Slow acting receptor that activates G-proteins when activated. AKA G-protein coupled receptor.
Ability of a molecule to bind to its receptor.
Membrane protein required for neurotransmitter signalling by metabotropic receptors. Involved in regulation of ion channels and effector enzyme synthesis or breakdown.
Substance that when activated initiates intracellular biochemical process.
Enzymes that catalyze the phosphorylation of other proteins.
Phenomenon where synaptic connections are strengthened. Requires the activation of NMDA and AMPA receptors.
Excitatory amino acid neurotransmitter.
Electrical stimuli delivered repeatedly that causes long term potentiation.