Brain, Spinal Cord and the Periphery
When you think of the nervous system, what comes to mind? Usually, our first thought is the brain and spinal cord. As important as these structures are, they only make up one part of the nervous system and its complex functions. In this subchapter, we will review the brain, spinal cord, and all the peripheral structures that make up what is known as the human nervous system. We will also cover the different cell types that are present in this area and their functions, so that we can start applying the concepts we have learned in the course thus far.
Learning Outcomes
In this section you will learn…
- The basic structure and function of the nervous system.
- The differences between neurons in the nervous system.
- The types of glial cells and their role in neurological function.
- The process of myelination by Schwann cells and oligodendrocytes respectively.
- How signals are communicated through a chemical synapse.
CNS and PNS[1]
The nervous system has two major regions: the central and peripheral nervous systems. The central nervous system (CNS) is made up of the brain and spinal cord. The brain is contained within the cranial cavity of the skull and the spinal cord is located within the vertebral cavity of the vertebral column. In contrast, the peripheral nervous system (PNS) refers to all structures of the nervous system located beyond the brain and spinal cord, that is, the periphery of the body.
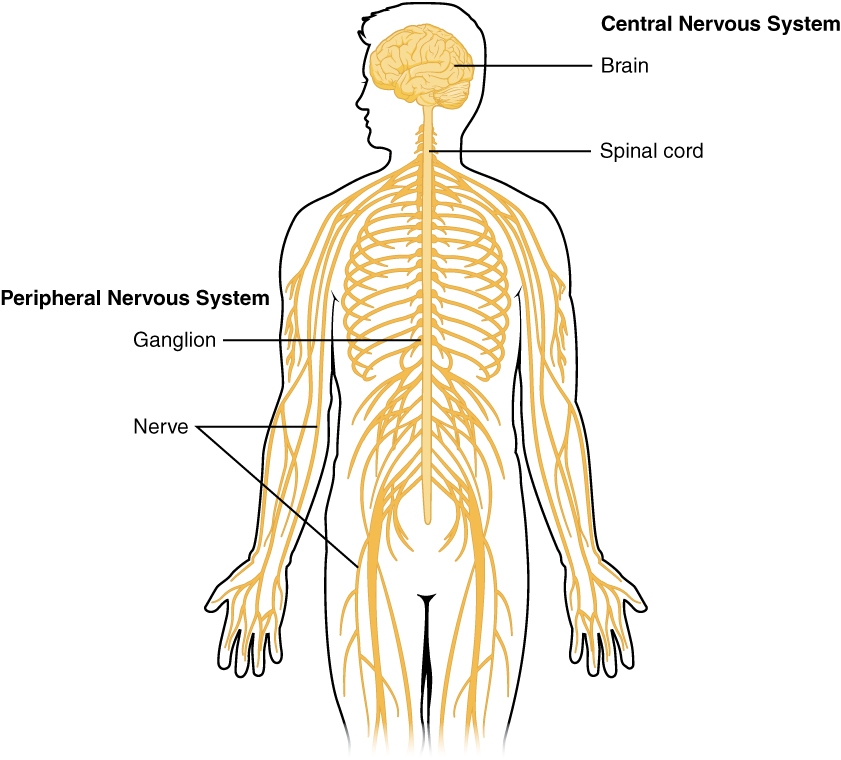
Cell Structure and Function
The CNS and PNS contain two basic types of cells: neurons and glial cells. Glial cells provide a framework of tissue that supports the neurons and their activities. The functional unit for communication within the nervous system is the neuron. It is comprised of the following the soma which is the cell body that has extensions called processes, axon which is an important process in every neuron that allows transmission of information, and dendrites which are an important process responsible for receiving input from other neurons. Some regions contain mostly cell bodies and other regions are largely just axons.
The Spinal Cord and the Brain[2]
Connecting to the brainstem and extending down the body through the spinal column is the spinal cord, shown in figure 1. The spinal cord is a thick bundle of nervous tissue that carries information from the body to the brain and from the brain to the body. The spinal cord is contained within the bones of the vertebral column and is able to communicate with the body through its connections with spinal nerves (part of the peripheral nervous system). A cross-section of the spinal cord looks like a white oval containing a grey butterfly-shape, as illustrated in figure 2. Myelinated axons make up the “white matter” and neuron and glial cell bodies make up the “grey matter.” Grey matter is also composed of interneurons, which connect two neurons each located in different parts of the body. Axons and cell bodies in the posterior spinal cord convey mostly sensory information from the body to the brain. Axons and cell bodies in the anterior spinal cord primarily transmit signals controlling movement from the brain to the body.
The spinal cord also controls motor reflexes. These reflexes are quick, unconscious movements—like automatically removing a hand from a hot object. Reflexes are so fast because they involve local synaptic connections. For example, the knee reflex that a doctor tests during a routine physical are controlled by a single synapse between a sensory neuron and a motor neuron. While a reflex may only involve one or two synapses, the brain is still informed of the event by interneurons in the spinal column (the knee jerked, or the hand was hot). The spinal cord is the information superhighway that connects the brain with the rest of the body through its connections with peripheral nerves. It transmits sensory and motor input and also controls motor reflexes.
Are you interested in learning more about spinal cord injuries? In the United States, there around 10,000 spinal cord injuries each year. Because the spinal cord is the information superhighway connecting the brain with the body, damage to the spinal cord can lead to paralysis. The extent of the paralysis depends on the location of the injury along the spinal cord and whether the spinal cord was completely severed. For example, if the spinal cord is damaged at the level of the neck, it can cause paralysis from the neck down, whereas damage to the spinal column further down may limit paralysis to the legs. Spinal cord injuries are notoriously difficult to treat because spinal nerves do not regenerate, although ongoing research suggests that stem cell transplants may be able to act as a bridge to reconnect severed nerves. Researchers are also looking at ways to prevent the inflammation that worsens nerve damage after injury. One such treatment is to pump the body with cold saline to induce hypothermia. This cooling can prevent swelling and other processes that are thought to worsen spinal cord injuries.
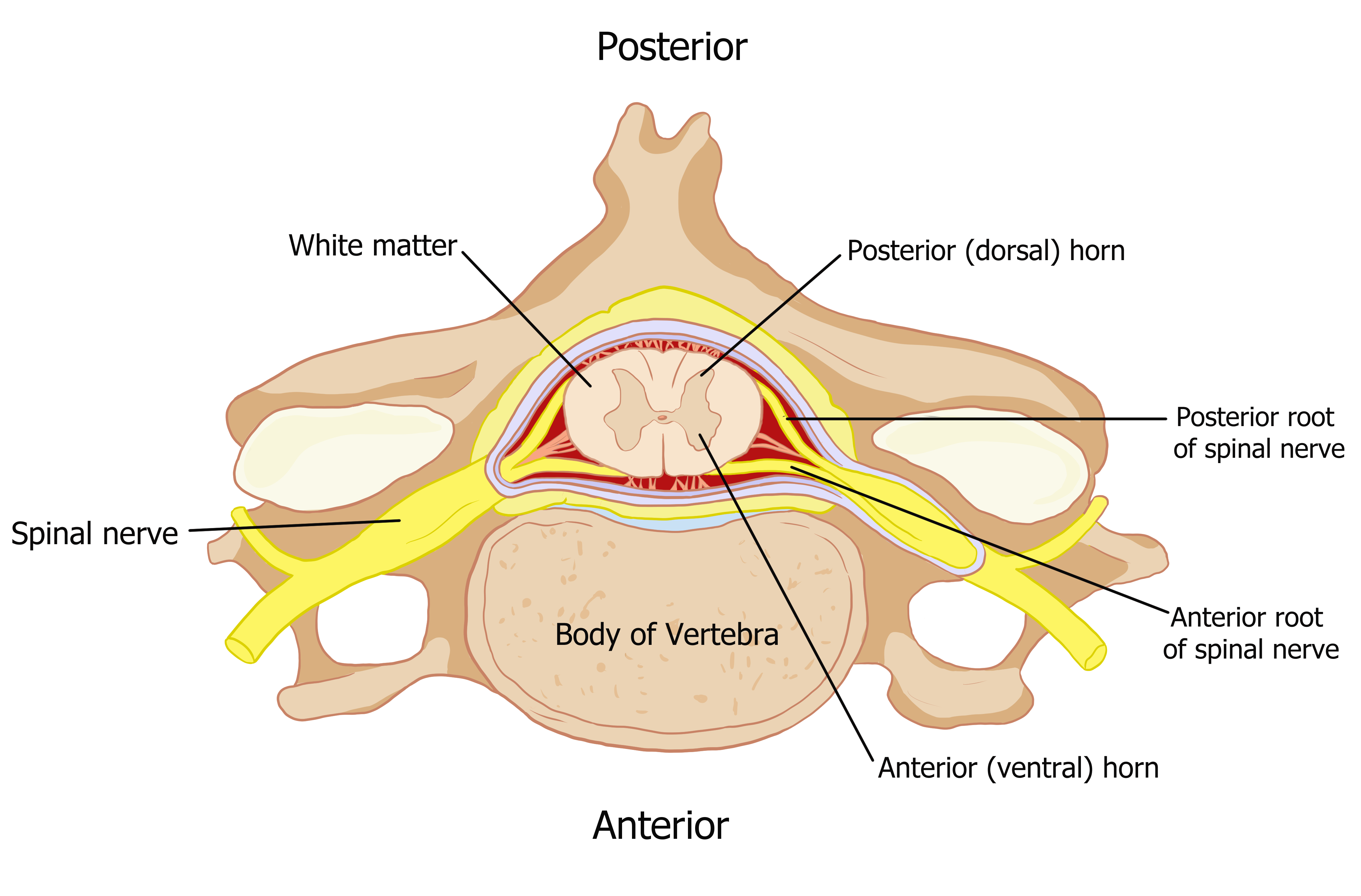
The vertebrate central nervous system contains the brain and the spinal cord, which are covered and protected by three meninges. The brain contains structurally and functionally defined regions. In mammals, these include the cortex (which can be broken down into four primary functional lobes: frontal, temporal, occipital, and parietal), basal ganglia, thalamus, hypothalamus, limbic system, cerebellum, and brainstem—although structures in some of these designations overlap. While functions may be primarily localized to one structure in the brain, most complex functions, like language and sleep, involve neurons in multiple brain regions.
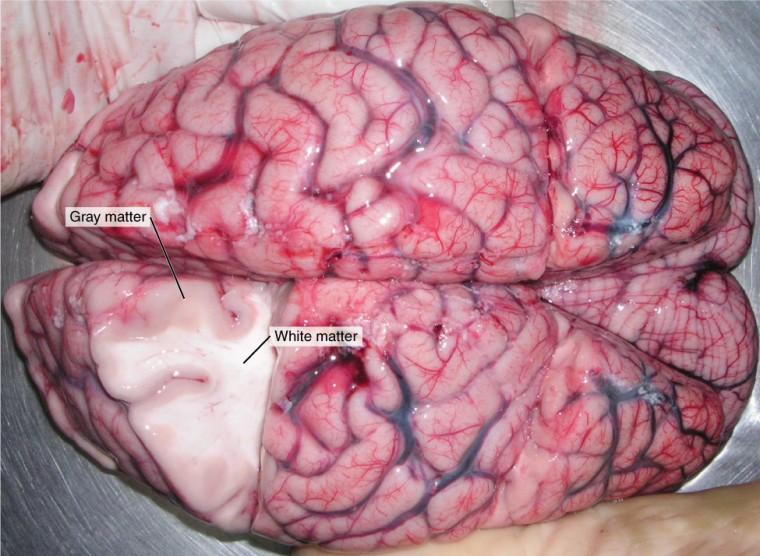
Ever wonder where the names grey matter and white matter come from? Grey matter is not necessarily grey, can be pinkish because of blood content. White matter is white because axons are insulated by a lipid-rich substance called myelin. Lipids can appear as white (“fatty”) material, much like the fat on a raw piece of chicken or beef. Actually, grey matter may have that colour ascribed to it because next to the white matter, it is just darker—hence, grey.
The Distinction: Nerve vs Tract
The terminology applied to bundles of axons differs depending on their location. Thetract is a bundle of axons, or fibers, found in the CNS. The nerve is a bundle of axons, or fibers found in the PNS.
Note: Both terms can be used to refer to the same bundle of axons. For example, the axons that project from the retina into the brain are called the optic nerve as they leave the eye, but when they are inside the cranium, they are referred to as the optic tract. There is a specific place where the name changes, which is the optic chiasm, but they are still the same axons.
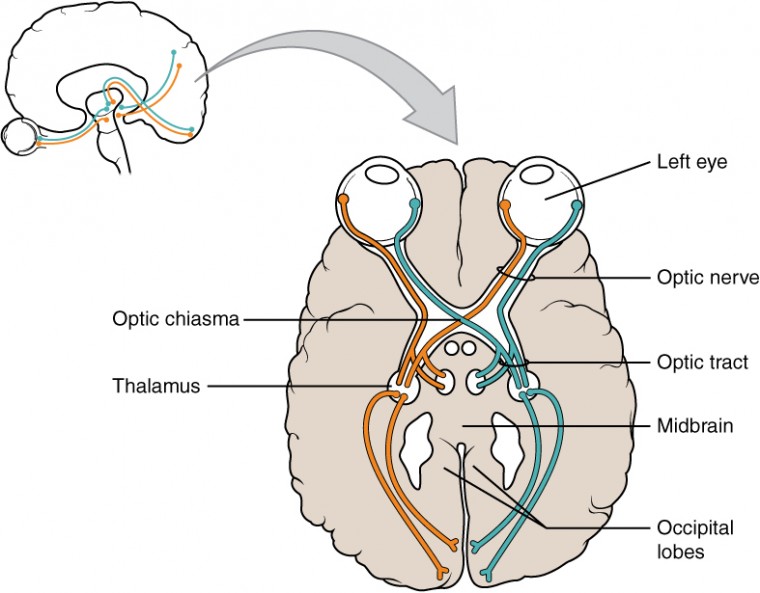
Are you interested in learning more about MRI technology? In 2003, the Nobel Prize in Physiology or Medicine was awarded to Paul C. Lauterbur and Sir Peter Mansfield for discoveries related to magnetic resonance imaging (MRI). This is a tool to see the structures of the body (not just the nervous system) that depends on magnetic fields associated with certain atomic nuclei. The utility of this technique in the nervous system is that fat tissue and water appear as different shades between black and white. Because white matter is fatty (from myelin) and grey matter is not, they can be easily distinguished in MRI images.
Nervous System: Basic Function
The nervous system is involved in receiving information about the environment around us and generating appropriate responses to that information. The nervous system can be divided into regions that are responsible for sensation (sensory functions) and for the response (motor functions). However, there is a third function that is required. Sensory input needs to be integrated with other sensations, as well as with memories, emotional state, and/or learning (cognition). The process of integration combines sensory perceptions and higher cognitive functions such as memories and emotion to produce a response. Some regions of the nervous system are deemed integration or association areas.
Sensation
The first major function of the nervous system is sensation—receiving information about the environment to gain input about what is happening outside the body (or within the body). The sensory functions of the nervous system register the presence of a change from homeostasis or a particular event in the environment, known as a stimulus.
The senses we think of most are the ‘big five’: taste, smell, touch, sight, and hearing. The stimuli for taste and smell are both chemical substances (molecules, compounds, ions, etc.), touch is physical or mechanical stimuli that interact with the skin, sight is light stimuli, and hearing is the detection of sound waves, which is a physical stimulus similar to some aspects of touch. Those five are all senses that receive stimuli from the outside world, and of which there is conscious perception. Additional sensory stimuli might be from the internal environment (inside the body), such as the stretch of an organ wall or the concentration of certain ions in the blood.
Response
The nervous system produces a response on the basis of the stimuli perceived by sensory structures. An obvious response would be the movement of muscles, such as withdrawing a hand from a hot stove, but there are broader uses of the term. The nervous system can cause the contraction of all three types of muscle tissue. For example, skeletal muscle contracts to move the skeleton, cardiac muscle is influenced as heart rate increases during exercise, and smooth muscle contracts as the digestive system moves food along the digestive tract. Responses also include the neural control of glands in the body, such as the production and secretion of sweat by the glands found in the skin in order to lower body temperature.
Responses can be divided into those that are voluntary or conscious (contraction of skeletal muscle) and those that are involuntary (contraction of smooth muscles, regulation of cardiac muscle, activation of glands). Voluntary responses are governed by the somatic nervous system and involuntary responses are governed by the autonomic nervous system.
Integration
Stimuli that are received by sensory structures are communicated to the nervous system where perception is formed in a process known as integration. Stimuli are integrated together, or with memories of previous stimuli, or the state of a person at a particular time. This leads to the specific response that will be generated. Seeing a baseball pitched to a batter will not automatically cause the batter to swing. The trajectory of the ball and its speed will need to be considered. Maybe the count is three balls and one strike, and the batter wants to let this pitch go by in the hopes of getting a walk to first base. Or maybe the batter’s team is so far ahead, it would be fun to just swing away.
Testing Your Knowledge
Thinking Beyond:
Let’s see how our knowledge regarding the nervous systems basic function helps us answer this question. Keeping the “big five” senses and their importance to survival in mind, why do you think perception and integration are so tightly linked to a response? Hint: Think about smell in an evolutionary sense, and why a bad stench was such important information to us.
Neurons[3]
There are many neurons in the nervous system—a number in the trillions. There is also a variety of different types of neurons. These neurons can be classified in a few ways. One way to classify them is by the number of processes attached to the cell body. Using the standard model of neurons, one of these processes is the axon, and the rest are dendrites. Because information flows through the neuron from dendrites or cell bodies toward the axon, these names are based on the neuron’s polarity.
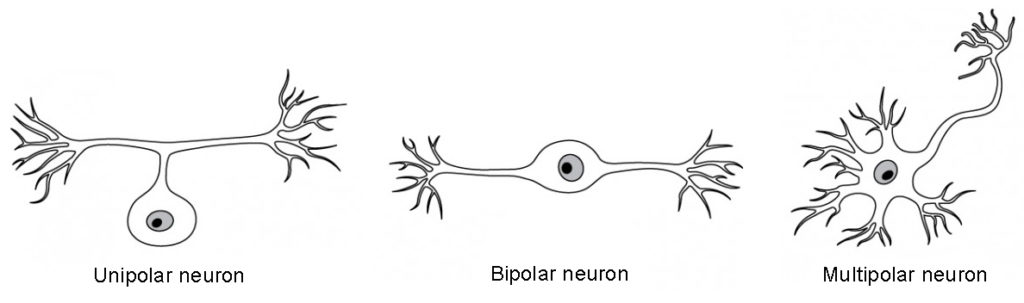
Unipolar
Unipolar cells have only one process emerging from the cell. True unipolar cells are only found in invertebrate animals, so in humans, they are more appropriately named “pseudo-unipolar” cells. Invertebrate unipolar cells do not have dendrites. Pseudo-unipolar cells have an axon that emerges from the cell body, splitting so that it can extend a great distance. At one end of the axon are dendrites, and at the other end, the axon forms synaptic connections with a target. Unipolar cells are exclusively sensory neurons and have two unique characteristics. First, their dendrites are receiving sensory information, sometimes directly from the stimulus itself. Secondly, the cell bodies of unipolar neurons are always found in ganglia. Sensory reception is a peripheral function (those dendrites are in the periphery) so the cell body is in the periphery, though closer to the CNS in a ganglion. The axon projects from the dendrite endings, past the cell body in a ganglion, and into the CNS.
Bipolar
Bipolar cells have two processes, which extend from each end of the cell body opposite each other. One is the axon and one the dendrite. Bipolar cells are not very common. They are found mainly in the olfactory epithelium (where smell stimuli are sensed), and as part of the retina.
Multipolar
Multipolar neurons are all of the neurons that are not unipolar or bipolar. They have one axon and two or more dendrites. With the exception of the unipolar sensory ganglion cells, and the two specific bipolar cells mentioned above, all other neurons are multipolar. Some cutting-edge research suggests that certain neurons in the CNS do not conform to the standard model of “one, and only one” axon. These sources describe a fourth type of neuron called an anaxonic neuron. The name suggests that it has no axon (an- = “without”), but this is not accurate. This is apparent when looking through a microscope at the standard resolution used in histology (approximately 400X to 1000X total magnification), as axons and dendrites are indistinguishable due to their small size. Any of these processes can function as an axon depending on the conditions. Nevertheless, even if they cannot be easily seen, and we do not know if one specific process is definitively the axon, these neurons have multiple processes and are therefore multipolar.
Communication Between Neurons
The dendrite extensions will receive neurotransmitter signals from upstream neurons that will either depolarize or hyperpolarize the neuron. This change in voltage will travel to the downstream neuron or effector cell along the axon. At the dendrites, excitatory and inhibitory neurotransmitters antagonize each other until the scales are tipped towards one side, which will result in depolarization or hyperpolarization of the cell body. This summation of positive and negative signals is what we call a graded potential, an example of this summation is illustrated in figure 6. As we can see there are more excitatory than inhibitory signals so the membrane potential rises from −70mV to −55mV: the threshold for the membrane which results in an action potential.
For receptor potentials, threshold is not a factor because the change in membrane potential for receptor cells directly causes neurotransmitter release. However, generator potentials can initiate action potentials in the sensory neuron axon, and postsynaptic potentials can initiate an action potential in the axons of other neurons. Graded potentials summate at a specific location at the beginning of the axon to initiate the action potential, namely the initial segment. For sensory neurons, which do not have a cell body between the dendrites and the axon, the initial segment is directly adjacent to the dendritic endings. For all other neurons, the axon hillock is essentially the initial segment of the axon, and it is where summation takes place. These locations have a high density of voltage-gated Na+ channels that initiate the depolarizing phase of the action potential.
Summation can be spatial or temporal, meaning it can be the result of multiple graded potentials at different locations on the neuron, or all at the same place but separated in time. Spatial summation is related to associating the activity of multiple inputs to a neuron with each other. Temporal summation is the relationship of subsequent action potentials from a single cell resulting in a significant change in the membrane potential. Spatial and temporal summation can also act together.
Tips From Past Students
Note that figure 6 is touching on one of the biggest themes of this unit: integration. The principle of summation is just one of the methods of integration in the body, so be sure to look over the other methods!
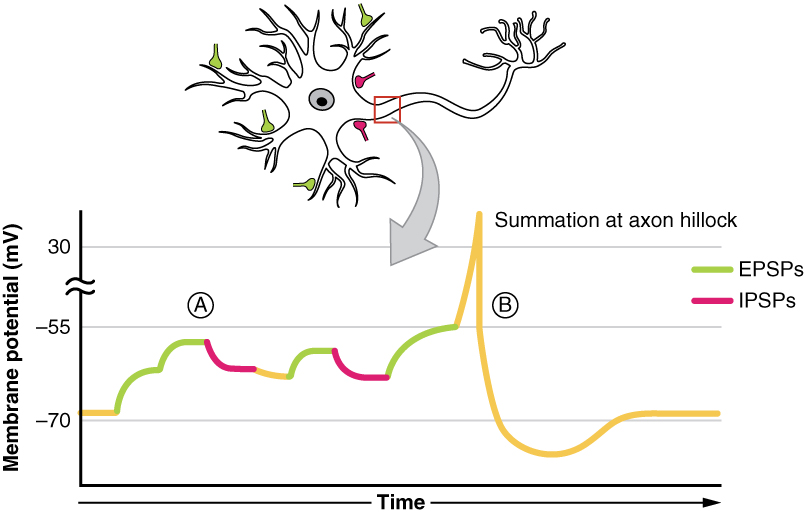
Testing Your Knowledge
Thinking Beyond:
Let’s see how our knowledge regarding excitable neurons can be applied to the following question. In what areas throughout the body would graded potentials such as these be more advantageous than Na+ leak channels and vice versa? Hint: Consider what areas of the body excitation may be more tightly regulated and what areas may require rhythmic activation.
Glial Cells
Glial cells, or neuroglia or simply glia, are the other type of cell found in nervous tissue. They are considered to be supporting cells, and many functions are directed at helping neurons communicate. The name glia comes from the Greek word meaning “glue,” and was coined by the German pathologist Rudolph Virchow, who wrote in 1856: “This connective substance, which is in the brain, the spinal cord, and the special sense nerves, is a kind of glue in which the nervous elements are planted.” Today, research into nervous tissue has shown that there are many deeper roles that these cells play. And research may find much more about them in the future.
There are six types of glial cells. Four of them are found in the CNS and two in the PNS. Table 1 outlines some common characteristics and functions. Astrocytes and Satellite cells are found in the CNS and PNS respectfully, and they provide support for the nervous system. Oligodendrocytes and Schwann cells, in the CNS and PNS respectively, both provide insulation by myelinating neuron axons. Microglia in the CNS will provide immune surveillance and perform phagocytosis. Finally, ependymal cells in the CNS will create cerebral spinal fluid.
Glial Cells of the CNS
One cell providing support to neurons of the CNS is the astrocyte, named so because it appears to be star-shaped under a microscope (astro– = “star”). Astrocytes have many processes extending from their main cell body (not axons or dendrites like neurons, just cell extensions). These processes extend to interact with neurons, blood vessels, or the connective tissue covering the CNS.
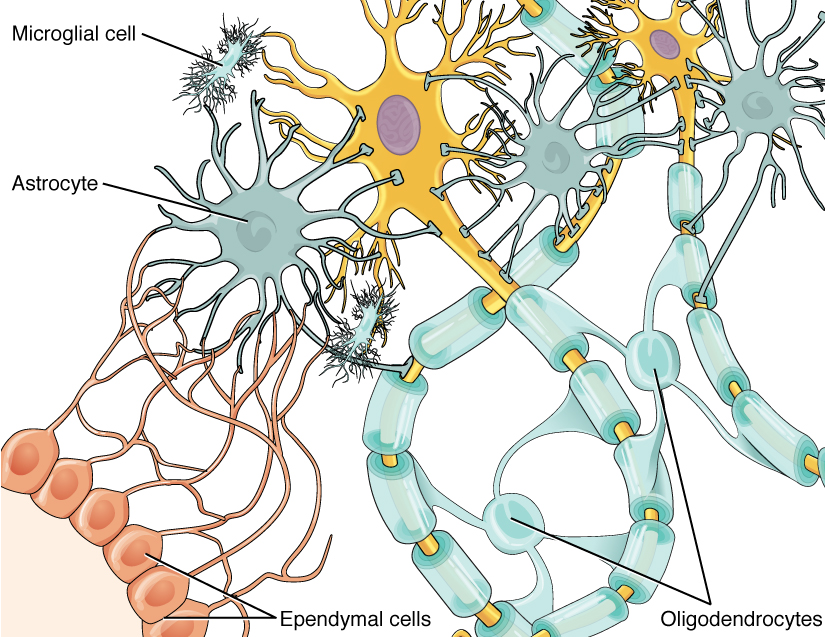
Generally, astrocytes are supporting cells for the neurons in the CNS. They accomplish this by maintaining the concentration of chemicals in the extracellular space, removing excess signaling molecules, reacting to tissue damage, and contributing to the blood-brain barrier (BBB). The BBB is a physiological barrier that keeps substances that circulate in the rest of the body from getting into the central nervous system, restricting what can cross from circulating blood.
Like a few other parts of the body, the brain has a privileged blood supply. Very little can pass through by diffusion. Most substances that cross the wall of a blood vessel into the CNS must do so through an active transport process. Because of this, only specific types of molecules can enter the CNS. Glucose—the primary energy source—is allowed, as are amino acids. Water and some other small particles, like gases and ions, can enter. But most everything else cannot, including white blood cells, which are one of the body’s main lines of defense. While the BBB protects the CNS from exposure to toxic or pathogenic substances, it also keeps out the cells that could protect the brain and spinal cord from disease and damage. The BBB also makes it harder for pharmaceuticals to be developed for the central nervous system.
Also found in nervous tissue is the oligodendrocyte, which is the glial cell type that insulates axons only in the CNS. The name means “cell of a few branches” (oligo– = “few”; dendro– = “branches”; –cyte = “cell”). There are multiple processes that extend from the cell body, each one reaching out and surrounding an axon to insulate it in myelin. One oligodendrocyte will provide the myelin for multiple axon segments, either for the same axon or for separate axons. The function of myelin will be discussed later in this chapter.
Microglia are the smallest of the glial cells. Ongoing research into these cells suggests that they may originate as white blood cells, called macrophages, that become part of the CNS during early development. While their origin is not conclusively determined, their function is related to their bodily macrophage counterparts. When macrophages encounter diseased or damaged cells in the rest of the body, they ingest and digest those cells or pathogens that cause disease. Microglia are the cells in the CNS that can do this in normal, healthy tissue, and they are therefore also referred to as CNS-resident macrophages.
The ependymal cell is a glial cell that filters blood to make cerebrospinal fluid (CSF), the fluid that circulates through the CNS. Because of the privileged blood supply inherent in the BBB, the extracellular space in nervous tissue does not easily exchange components with the blood. Ependymal cells line each of the brain’s four ventricles, which are hollow cavities in the brain that contain CSF. The choroid plexus is a specialized structure in the ventricles where ependymal cells come into contact with blood vessels, filtering and absorbing components of the blood to produce cerebrospinal fluid.
Because of this, ependymal cells can be considered a component of the BBB, or a place where the BBB is thin. These glial cells function similarly to epithelial cells, making a single layer of cells with little intercellular space and tight connections between adjacent cells. They also have cilia on their apical surface to help move the CSF through the ventricular space. The relationship of these glial cells to the structure of the CNS is seen in figure 8.
Testing Your Knowledge
Thinking Beyond:
Let’s see how our knowledge of the CNS in contrast to the PNS helps us answer this question. Oligodendrocytes are associated with multiple neurons and are found only in the CNS, whereas Schwann cells are associated with a single neuron and are found in the PNS. Why do you think this relationship occurs? Hint: Consider figure 1 to see the volume of neurons in the two systems.
Glial Cells of the PNS
One of the two types of glial cells found in the PNS is the satellite cell. Satellite cells are found in sensory and autonomic ganglia, where they surround the cell bodies of neurons. They provide support, performing similar functions in the periphery as astrocytes do in the CNS—except, of course, for establishing the BBB.
The second type of glial cell is the Schwann cell, which insulates axons with myelin in the periphery. Schwann cells are different from oligodendrocytes, in that a Schwann cell wraps around a portion of only one axon segment whereas oligodendrocytes have processes that reach out to multiple axon segments. The nucleus and cytoplasm of the Schwann cell are on the edge of the myelin sheath. The relationship of these two types of glial cells to ganglia and nerves in the PNS is seen in figure 5.
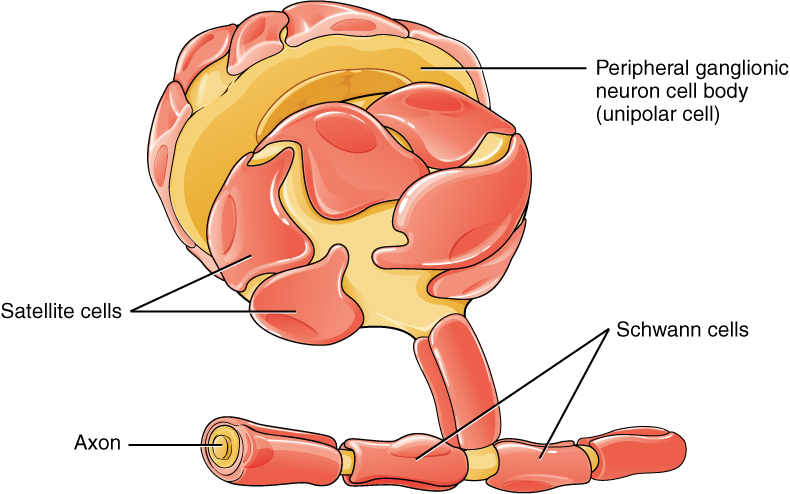
Myelin
The insulation for axons in the nervous system is provided by glial cells; oligodendrocytes in the CNS, and Schwann cells in the PNS. These cells provide insulation by producing and coating the axons of neurons with myelin. Myelin is a lipid-rich layer that surrounds the axon creating a myelin sheath that facilitates the transmission of electrical signals along the axon.
The appearance of the myelin sheath can be thought of as similar to the pastry wrapped around a hot dog for “pigs in a blanket” or a similar food. The glial cell is wrapped around the axon several times with little to no cytoplasm between the glial cell layers. For oligodendrocytes, as seen in figure 8, the cell body resides away from the neurons. For each oligodendrocyte cell body, there are processes extending to myelinate several segments of multiple axons. For Schwann cells, as seen in figure 9, the outermost layer of the cell membrane contains cytoplasm. The nucleus of the Schwann cell bulges out on one side.
The myelin sheath is not inherent to the neuron. Myelin is produced by non-neuronal glial cells which provide support for the nervous system. Glial function also includes holding neurons in place, supplying them with nutrients, and removing pathogens and dead neurons. Recall that in the central nervous system, the glial cells that form the myelin sheath are called oligodendrocytes; in the peripheral nervous system, they are called Schwann cells.
During development, the glial cell is loosely or incompletely wrapped around the axon, as seen in figure 10a. The edges of this loose enclosure extend toward each other, and one end tucks under the other. The inner edge wraps around the axon, creating several layers, and the outer edge closes around the outside so that the axon is completely enclosed in the myelin layer.
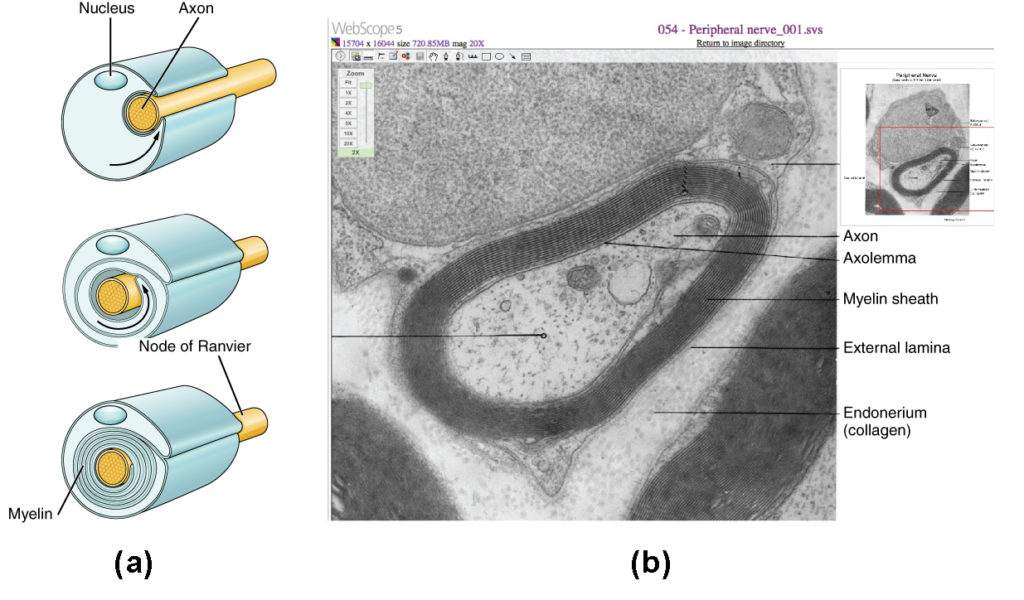
Are you interested in learning about diseases associated with demyelination? Several diseases can result from the demyelination of axons. The causes of demyelination include genetic factors, pathogens, and autoimmune disorders. Though the causes are varied, the results are largely similar. The myelin insulation of axons is compromised, making electrical signaling slower.
Multiple sclerosis (MS) is one such disease. It is an example of an autoimmune disease. The antibodies produced by lymphocytes (a type of white blood cell) mark myelin as something that should not be in the body. This causes inflammation and the destruction of the myelin in the central nervous system. As the insulation around the axons is destroyed by the disease, scarring becomes obvious. This is where the name of the disease comes from; sclerosis means hardening of tissue, which is what a scar is. Multiple scars are found in the white matter of the brain and spinal cord. The symptoms of MS include both somatic and autonomic deficits. Control of the musculature is compromised, as is control of organs such as the bladder.
Guillain-Barré syndrome is an example of demyelinating diseases of the peripheral nervous system. It is also the result of an autoimmune reaction, but the inflammation is in peripheral nerves. Sensory symptoms or motor deficits are common, and autonomic failures can lead to changes in the heart rhythm or a drop in blood pressure, especially when standing, which causes dizziness.
Nervous Tissue
Nervous tissue is made up of neurons and glial cells. Neurons are electrically active and send chemical signals to target cells. This is the main method of communication that the nervous system utilizes.
Neural Impulses in the Nervous System
The nervous system goes through a three-step process when it functions: sensory input, neural processing, and motor output. The sensory input stage is when the neurons (or excitable nerve cells) of the sensory organs are excited by stimuli. Neural impulses from sensory receptors are sent to the brain and spinal cord for processing. After the brain has processed this information, neural impulses are conducted from the brain and spinal cord to muscles and glands, resulting in motor output.
A neuron communicates with other neurons by releasing a neurotransmitter that binds to chemical receptors. The effect upon the postsynaptic (receiving) neuron is determined not by the presynaptic (sending) neuron or by the neurotransmitter itself, but by the type of receptor that is activated. A neurotransmitter can be thought of as a key, and a receptor as a lock: the key unlocks a certain response in the postsynaptic neuron, communicating a particular signal. However, in order for a presynaptic neuron to release a neurotransmitter to the next neuron in the chain, it must go through a series of changes in electric potential.
Sensory Nerves
Once any sensory cell transduces a stimulus into a nerve impulse, that impulse has to travel along axons to reach the CNS. In many of the special senses, the axons leaving the sensory receptors have a topographical arrangement, meaning that the location of the sensory receptor relates to the location of the axon in the nerve. For example, in the retina, axons from retinal glial cells (RGCs) in the fovea are located at the center of the optic nerve, where they are surrounded by axons from the more peripheral RGCs.
Spinal Nerves
Generally, spinal nerves contain afferent axons from sensory receptors in the periphery mixed with efferent axons traveling to the muscles or other effector organs. As the spinal nerve nears the spinal cord, it splits into posterior and anterior roots. The posterior root contains only the axons of sensory neurons, whereas the anterior roots contain the axons of the motor neurons. Some branches will synapse with local neurons in the posterior root ganglion, the posterior horn, or the anterior horn, at the level of the spinal cord where they enter. Others will travel a short distance up or down the spine to interact with neurons at different vertebral levels. A branch may also turn into the posterior column of the white matter to connect with the brain. Typically, spinal nerve systems that connect to the brain are contralateral, meaning that the right side of the body is connected to the left side of the brain and the left side of the body connects to the right side of the brain.
The Chemical Synapse[4]
The electric signal of an action potential involves the release of a signaling molecule that binds to a receptor protein on a target cell. This is triggered by a synapse, which can either be adrenergic or cholinergic. Adrenergic synapses release norepinephrine and cholinergic release acetylcholine. These signaling molecules bind to different receptors.
The adrenergic system has two types of G-protein coupled receptors: the alpha and beta-adrenergic receptors. The cholinergic system also has two types of receptors: the nicotinic receptor and muscarinic receptors. The nicotinic receptor is a ligand-gated cation channel, whereas the muscarinic receptor is a G protein-coupled receptor. Although these receptors are structurally and functionally distinct they are called cholinergic receptors because the “master key” for both is acetylcholine (Ach). Thinking of cholinergic receptors as two locks, one for a classroom door and the other for an office door, both doors will have their own specific key, with a master key to open both. As such, nicotinic and muscarinic receptors can be activated by the exogenous drugs nicotine and muscarine respectively, with Ach as the primary ligand for both.
Tips From Past Students
This is one of the foundational topics of physiology that is crucial to understanding not only this course but the greater field of biology. Know each step and be able to explain what is happening. If you understand the process, it will be significantly easier to replicate on an exam.
The process of a chemical reaction at the synapse has some important differences from an electrical reaction. Chemical synapses are much slower than electrical synapses because neurotransmitters must diffuse through the synaptic cleft to bind to a receptor and elicit a response. Like electrical reactions, chemical reactions involve electrical modifications at the postsynaptic membrane, which is facilitated by neurotransmitters that are released from the upstream neuron.
A basic chemical reaction at the synapse undergoes a few additional steps:
- The action potential (which occurs as described above) travels along the membrane of the presynaptic cell until it reaches the synapse. The electrical depolarization of the membrane at the synapse causes voltage-gated calcium (Ca2+) channels to open.
- The ions flow through the presynaptic cell membrane, rapidly increasing their concentration in the interior.
- This high concentration activates a set of ion-sensitive proteins attached to vesicles, which are small membrane compartments that contain a neurotransmitter chemical.
- These proteins change shape, causing the membranes of some “docked” vesicles to fuse with the membrane of the presynaptic cell. This opens the vesicles, which releases their neurotransmitter contents into the synaptic cleft, the narrow space between the membranes of the pre- and postsynaptic cells.
- The neurotransmitter diffuses into the cleft. Some of it escapes, but the rest binds to chemical receptors located on the membrane of the postsynaptic cell.
- The binding of neurotransmitters causes the receptor molecule to be activated in some way. Several types of activation are possible, depending on what kind of neurotransmitter is binding. In any case, this is the key step by which the synaptic process causes electrical modification of the postsynaptic cell.
- The neurotransmitter is either reabsorbed by the presynaptic cell and repackaged for future release, or it is broken down metabolically.
Key Terms Exercise
Key Takeaways
Consider the following concepts to help guide your studies:
- Neurons are specialized cells that transmit chemical and electrical signals in the brain and serve as communicators between sensory receptors, muscles and glands, and the brain and spinal cord; they are the functional units of the central nervous system.
- There are three basic types of neurons: unipolar (one projection), bipolar (two projections), and multipolar (more than two projections). Projections are either the axon or the dendrites.
- Sensory neurons are responsible for converting external stimuli from the environment into electrical signals.
- Motor neurons originate in the CNS; they project their axons outside of the CNS to directly or indirectly control muscles.
- Interneurons act as a bridge between sensory and motor neurons.
- Glial cells are the glue that holds the nervous system together and acts to support neurons.
- Astrocytes, oligodendrocytes, microglia, and ependymal cells are Glial cells found in the CNS, neurons in PNS are provided support by satellite cells and Schwann cells.
- The chemical synapse is a slower alternative to electrical synapse communication between neurons. The process involves the presynaptic neuron releasing neurotransmitters that will modulate the membrane potential of the postsynaptic neuron.
Subchapter Quiz
The questions below can be used to assess your knowledge within this chapter. There are five multiple-choice questions that you should attempt without referring to your notes. The questions will provide you with responses to your answers to guide your studying but should not be used as your only resource.
Media Attributions
- Central Nervous System © OpenStax College is licensed under a CC BY (Attribution) license
- Spinal Cord In Vivo © Poorna Marla is licensed under a CC0 (Creative Commons Zero) license
- Brain Specimen © OpenStax College is licensed under a CC BY (Attribution) license
- Optic Chiasm © OpenStax College is licensed under a CC BY (Attribution) license
- Types of Neurons © OpenStax College is licensed under a CC BY (Attribution) license
- Postsynaptic Potential Summation © OpenStax College is licensed under a CC BY (Attribution) license
- Private: Parts of a Neuron © OpenStax College is licensed under a CC BY (Attribution) license
- Glial Cells of CNS © OpenStax College is licensed under a CC BY (Attribution) license
- Glial Cells of PNS © OpenStax College is licensed under a CC BY (Attribution) license
- Embryonic Myelination © OpenStax College is licensed under a CC BY (Attribution) license
- Private: Neuron-to-neuron communication © Wikipedia is licensed under a Public Domain license
- OpenStax College (25 April 2013). 12.1 Basic Structure and Function of the Nervous System. Retrieved April 10, 2021 from https://openstax.org/books/anatomy-and-physiology/pages/12-1-basic-structure-and-function-of-the-nervous-system ↵
- OER Commons (n.d.). The Central Nervous System. Retrieved April 10, 2021 from https://www.oercommons.org/courseware/module/15117/overview ↵
- OpenStax College (n.d.). Vision. Retrieved April 10, 2021 from https://openstax.org/books/anatomy-and-physiology/pages/12-2-nervous-tissue ↵
- OpenStax College (n.d.). Divisions of the Autonomic Nervous System. Retrieved April 10, 2021 from https://courses.lumenlearning.com/suny-ap1/chapter/divisions-of-the-autonomic-nervous-system/ ↵
Multiple projections from a body (soma) that includes dendrites and an axon.
A display of the inside or centre of an object by making a straight incision through the object at any angle.
Space between two cells, consisting of the pre-synaptic neuron, synaptic cleft, and the post-synaptic neuron. Involved in the transmission of information between two cells.
The anterior lobe of the cerebral cortex that is involved in voluntary movement, language, and executive function (higher level thinking).
Bilateral lobes found behind the ears. They are responsible for processing auditory information and for forming and storing memories.
The most posterior lobe which functions to process visual information in order to perceive what we see.
Lobe located medial and superior in the cerebral cortex. The functions are to integrate sensory information from several locations, help plan how to interact with objects and to distinguish where objects are in space.
Any collection of neuron axons that are found in the CNS.
Any collection of neuron axons that are found in the PNS.
The crossing of the optic tract from the left eye that is heading to the right lateral geniculate nucleus with the optic tract from the right eye that is heading to the left lateral geniculate nucleus.
Sensory information from any form of stimuli that is received from the body.
The reactionary action undergone by the body following the perception of stimuli.
The combination of signals, both excitatory and inhibitory, from various origins that will influence the action or inaction of the body.
A detectable change in the physical or chemical structure of an organism's internal or external environment.
The aspect of the nervous system that is voluntarily controlled, therefore actions are carried out by stimulating skeletal muscle to contract.
Involuntary regulation of glands and organs in the body.
A neuron in the path of the travelling signal that is further from the source than the neuron currently carrying the signal.
For chemical (NT) signals there is not a one-to-one signal-to-action potential, several excitatory and inhibitory signals add together. In order to get an action potential there must be enough excitatory signals to outweigh the inhibitory signals to cause depolarization.
The modulation of membrane potential of an excitable cell by the integration of several excitatory or inhibitory signals.
The summation of inputs that modulate a graded potential that come from different pre synaptic neurons.
The summation of inputs that subsequently occur following the current spatial summating signals.
A type of glial cell that appears star shaped and acts to support neurons in the central nervous system. They are the most abundant cell type in the CNS.
A barrier between circulating blood and the CNS that is highly selective, allowing nutrients and small products to reach the brain while preventing damage and infection by blocking diffusion of larger products and pathogens.
A type of glial cell that myelinate neurons in the CNS.
A type of glial cell that acts as the immune defense of the CNS, as macrophages.
A type of glial cell that lines the ventricles of the CNS and act to produce and regular cerebrospinal fluid.
A clear, colourless fluid found within the CNS which circulates nutrients and chemicals into and waste out of the brain. Additionally, it acts as a shock absorber by cushioning the brain and spinal cord.
The surface of an epithelial cell that is exposed to the body cavity or the exterior surface of an organ.
A type of glial cell found in the PNS that provide protection and nutrient support to neurons.
A type of glial cell that myelinate neurons in the PNS.
A layer of fatty tissue that surrounds the axons of neurons leading to faster neural communication.
The mechanism by which sensory information is converted into motor output by taking information from sensory receptors through action potentials and relaying it to appropriate centers in the brain, who then send our signals to create the appropriate response.
The means of communication between nerve cells where electrical signals are transmitted along a nerve due to internal or external stimuli.
A representation of the locations of organs/receptors and their direct relation to other parts of the body.
A small pit in the retina of the eye that is necessary for sharp central vision in actions such as reading. Light rays in this area fall directly on cone cells.
Relating to the side of the body opposite to where the signal/organ resides. Ex. Left brain controls right limb motor movements.
[of a receptor] Membrane receptor proteins that can bind epinephrine and norepinephrine.
A type of membrane receptor with 7 transmembrane domains that can be activated by multiple ligands, leading to the conversion of GDP into GTP and activation of a signalling cascade.
[of a receptor] Membrane receptor proteins that can bind acetylcholine.
Being caused by external factors.